Featured Topics
Featured series.
A series of random questions answered by Harvard experts.

Explore the Gazette
Read the latest.
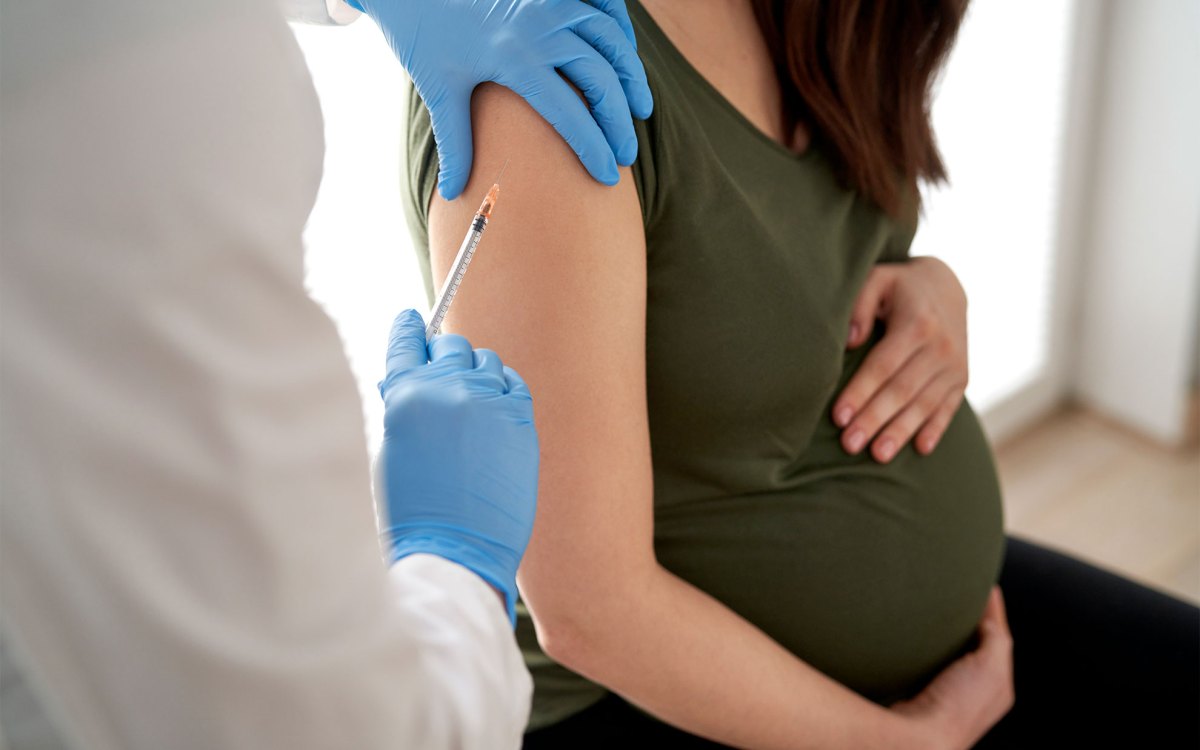
Study pinpoints optimal timing for RSV vaccine during pregnancy
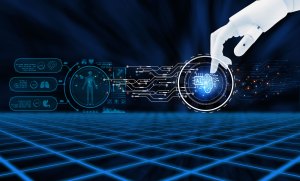
Cutting through the fog of long COVID

Warning for younger women: Be vigilant on breast cancer risk
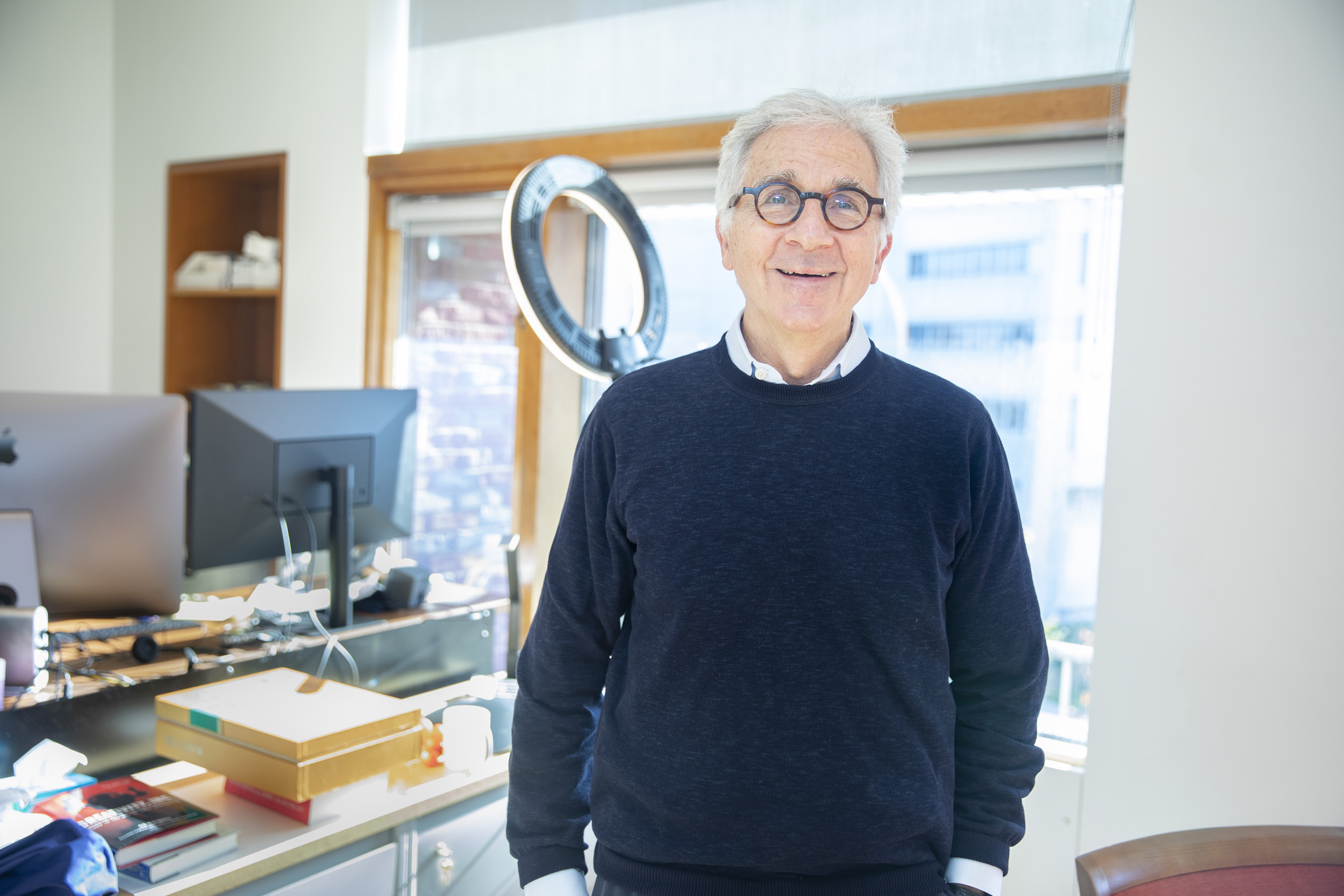
“When my son was diagnosed [with Type 1], I knew nothing about diabetes. I changed my research focus, thinking, as any parent would, ‘What am I going to do about this?’” says Douglas Melton.
Kris Snibbe/Harvard Staff Photographer
Breakthrough within reach for diabetes scientist and patients nearest to his heart
Harvard Correspondent
100 years after discovery of insulin, replacement therapy represents ‘a new kind of medicine,’ says Stem Cell Institute co-director Douglas Melton, whose children inspired his research
When Vertex Pharmaceuticals announced last month that its investigational stem-cell-derived replacement therapy was, in conjunction with immunosuppressive therapy, helping the first patient in a Phase 1/2 clinical trial robustly reproduce his or her own fully differentiated pancreatic islet cells, the cells that produce insulin, the news was hailed as a potential breakthrough for the treatment of Type 1 diabetes. For Harvard Stem Cell Institute Co-Director and Xander University Professor Douglas Melton, whose lab pioneered the science behind the therapy, the trial marked the most recent turning point in a decades-long effort to understand and treat the disease. In a conversation with the Gazette, Melton discussed the science behind the advance, the challenges ahead, and the personal side of his research. The interview was edited for clarity and length.
Douglas Melton
GAZETTE: What is the significance of the Vertex trial?
MELTON: The first major change in the treatment of Type 1 diabetes was probably the discovery of insulin in 1920. Now it’s 100 years later and if this works, it’s going to change the medical treatment for people with diabetes. Instead of injecting insulin, patients will get cells that will be their own insulin factories. It’s a new kind of medicine.
GAZETTE: Would you walk us through the approach?
MELTON: Nearly two decades ago we had the idea that we could use embryonic stem cells to make functional pancreatic islets for diabetics. When we first started, we had to try to figure out how the islets in a person’s pancreas replenished. Blood, for example, is replenished routinely by a blood stem cell. So, if you go give blood at a blood drive, your body makes more blood. But we showed in mice that that is not true for the pancreatic islets. Once they’re removed or killed, the adult body has no capacity to make new ones.
So the first important “a-ha” moment was to demonstrate that there was no capacity in an adult to make new islets. That moved us to another source of new material: stem cells. The next important thing, after we overcame the political issues surrounding the use of embryonic stem cells, was to ask: Can we direct the differentiation of stem cells and make them become beta cells? That problem took much longer than I expected — I told my wife it would take five years, but it took closer to 15. The project benefited enormously from undergraduates, graduate students, and postdocs. None of them were here for 15 years of course, but they all worked on different steps.
GAZETTE: What role did the Harvard Stem Cell Institute play?
MELTON: This work absolutely could not have been done using conventional support from the National Institutes of Health. First of all, NIH grants came with severe restrictions and secondly, a long-term project like this doesn’t easily map to the initial grant support they give for a one- to three-year project. I am forever grateful and feel fortunate to have been at a private institution where philanthropy, through the HSCI, wasn’t just helpful, it made all the difference.
I am exceptionally grateful as well to former Harvard President Larry Summers and Steve Hyman, director of the Stanley Center for Psychiatric Research at the Broad Institute, who supported the creation of the HSCI, which was formed specifically with the idea to explore the potential of pluripotency stem cells for discovering questions about how development works, how cells are made in our body, and hopefully for finding new treatments or cures for disease. This may be one of the first examples where it’s come to fruition. At the time, the use of embryonic stem cells was quite controversial, and Steve and Larry said that this was precisely the kind of science they wanted to support.
GAZETTE: You were fundamental in starting the Department of Stem Cell and Regenerative Biology. Can you tell us about that?
MELTON: David Scadden and I helped start the department, which lives in two Schools: Harvard Medical School and the Faculty of Arts and Science. This speaks to the unusual formation and intention of the department. I’ve talked a lot about diabetes and islets, but think about all the other tissues and diseases that people suffer from. There are faculty and students in the department working on the heart, nerves, muscle, brain, and other tissues — on all aspects of how the development of a cell and a tissue affects who we are and the course of disease. The department is an exciting one because it’s exploring experimental questions such as: How do you regenerate a limb? The department was founded with the idea that not only should you ask and answer questions about nature, but that one can do so with the intention that the results lead to new treatments for disease. It is a kind of applied biology department.
GAZETTE: This pancreatic islet work was patented by Harvard and then licensed to your biotech company, Semma, which was acquired by Vertex. Can you explain how this reflects your personal connection to the research?
MELTON: Semma is named for my two children, Sam and Emma. Both are now adults, and both have Type 1 diabetes. My son was 6 months old when he was diagnosed. And that’s when I changed my research plan. And my daughter, who’s four years older than my son, became diabetic about 10 years later, when she was 14.
When my son was diagnosed, I knew nothing about diabetes and had been working on how frogs develop. I changed my research focus, thinking, as any parent would, “What am I going to do about this?” Again, I come back to the flexibility of Harvard. Nobody said, “Why are you changing your research plan?”
GAZETTE: What’s next?
MELTON: The stem-cell-derived replacement therapy cells that have been put into this first patient were provided with a class of drugs called immunosuppressants, which depress the patient’s immune system. They have to do this because these cells were not taken from that patient, and so they are not recognized as “self.” Without immunosuppressants, they would be rejected. We want to find a way to make cells by genetic engineering that are not recognized as foreign.
I think this is a solvable problem. Why? When a woman has a baby, that baby has two sets of genes. It has genes from the egg, from the mother, which would be recognized as “self,” but it also has genes from the father, which would be “non-self.” Why does the mother’s body not reject the fetus? If we can figure that out, it will help inform our thinking about what genes to change in our stem cell-derived islets so that they could go into any person. This would be relevant not just to diabetes, but to any cells you wanted to transplant for liver or even heart transplants. It could mean no longer having to worry about immunosuppression.
Share this article
You might like.
Five weeks before giving birth best transfers maternal antibodies to the fetus, say researchers

Researchers say new AI tool sharpens diagnostic process, may help identify more people needing care
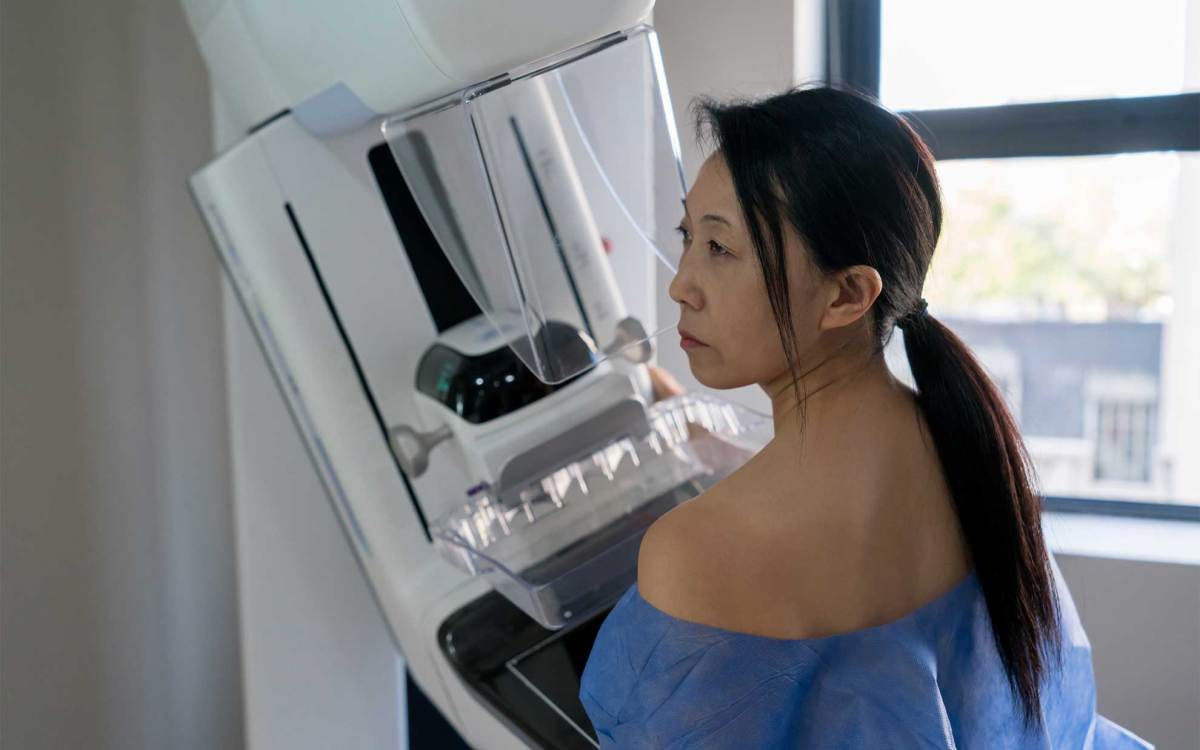
Pathologist explains the latest report from the American Cancer Society
U.S. fertility rates are tumbling, but some families still go big. Why?
It’s partly matter of faith. Economist examines choice to have large families in new book.
Did Trump election signal start of new political era?
Analysts weigh issues, strategies, media decisions at work in contest, suggest class may become dominant factor
Are optimists the realists?
Humanity is doing better than ever yet it often doesn’t seem that way. In podcast, experts make the case for fact-based hope.
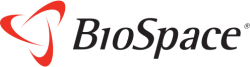
GIOSTAR Announces FDA Clearance of the IND for Starting PHASE-2 Clinical Trial for Developing Specific Stem Cell Therapy for Type II Diabetes.
SAN DIEGO--(BUSINESS WIRE)-- Global Institute of Stem Cell Therapy and Research, Inc. known as GIOSTAR , a San Diego, California based Global organization at the forefront of stem cell research over two decades, developing a novel cellular therapy pipeline to improve the standard of care for treating Type II diabetes patients, today announced that the United States Food and Drug Administration (FDA) has cleared its investigational new drug (IND) application to start a Phase–2 clinical trial for DT2-SCT. The Company’s novel approach using autologous mesenchymal stem cells to alleviate the disease-caused damage in diabetics offers a new hope to address the sufferings in diabetes patients without much side-effects.

Diabetes is not just a disease but a global health crisis. It is estimated to affect more than one billion people worldwide. The financial burden on the global healthcare system to treat diabetes is expected to reach more than one trillion dollars annually. GIOSTAR CEO, President, and Cofounder Mr. Deven Patel stated, “Upon a successful completion of the clinical trials, GIOSTAR intends to make this treatment affordable to masses and poised to capture significant global market share due to GIOSTAR’s existing global infrastructure of hospitals and research centers.”
According to the Chairman and Cofounder of GIOSTAR, Dr. Anand Srivastava , “DT2-SCT is a cellular therapy for Type II diabetics which uses autologous stem cells, isolated from the visceral tissues of the recipients, developed to target systemic ill-effects caused by diabetes-induced pathology in patients. We are pleased to reach this milestone following extensive research and development.”
GIOSTAR expects to complete the Phase-2 clinical trial using the DT2-SCT in Type II diabetics within 12 to 18 months. GIOSTAR anticipates enrolling participants for the study at few sites across the United States.
“The diabetes is now considered as a lifestyle disease. In many cases, even lifestyle changes are not enough to eliminate the risk of developing diabetes due to certain genetic risk factors,” stated Patel . “Our innovative and noninvasive stem cell-based therapeutics may offer better treatment option for diabetic patients.”
About: ( GIOSTAR )
GIOSTAR is a global stem cell research organization committed to the discovery, development, and commercialization of stem cell based treatments to make a meaningful difference in the lives of people impacted by difficult-to-treat degenerative and other diseases. The company also aims to develop stem cell-based therapies for arthritis, long COVID complications , cancer vaccines and the fairly uncommon area of making red blood cells from stem cells. GIOSTAR red blood cell technology is ready to scale and preparing its IND for US FDA. The leadership of GIOSTAR aims to make these stem cell treatments available to the masses at affordable prices.
Investor & Media Inquiries: Mr. Deven Patel CEO, President and Cofounder Global Institute Of Stem Cell Therapy And Research [email protected] www.giostar.com
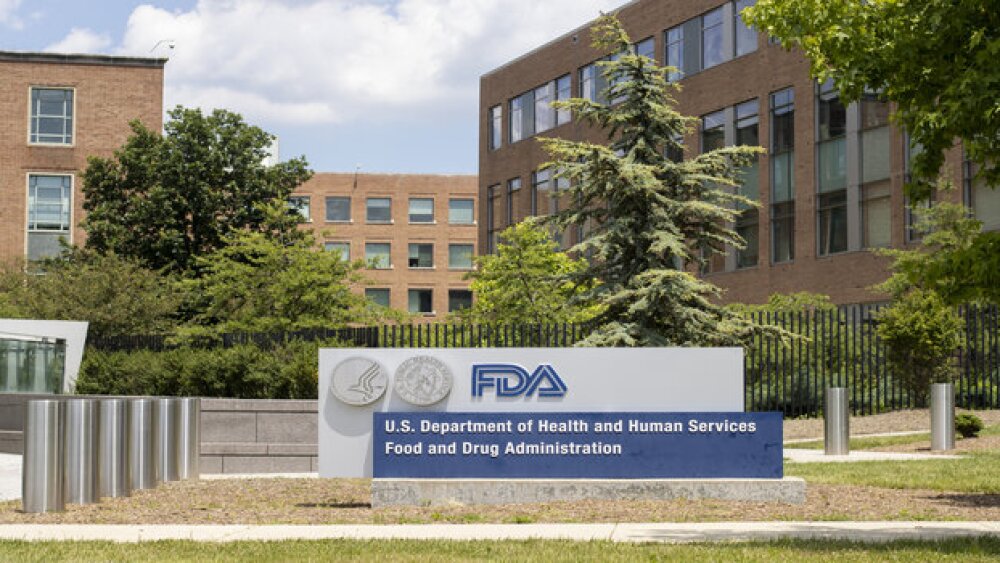
Talk to us about diabetes
0345 123 2399
customer support
Smart insulin and stem cell transplants: Research Highlights October 2024

We take a look at some of the exciting diabetes research developments announced in October, and what the findings could mean for people living with or affected by diabetes.
In this month's article:
- A step forward in making insulin 'smarter'
- World-first stem cell transplant helps person with type 1 diabetes produce insulin
- Regenerating cells with electrical impulses in people with type 2 diabetes
- New driver of diabetic retinopathy discovered
A step forward in making insulin ‘smarter’
A new type of ‘smart’ insulin has shown promise in early-stage research. In animals, the insulin was found to only turn on when blood sugar levels are high and stay off when levels are low.
Millions of people with all types of diabetes in the UK use insulin to manage their blood sugar levels. This requires a careful balancing act and it’s difficult to get right. So, scientists are trying to develop cleverer insulins that can sense blood sugar levels and respond in the right way. This would make life for people with diabetes dramatically simpler.
In new research published in Nature , an international research team tested a new type of ‘smart’ insulin they’ve developed, called NNC2215. It has a special molecular switch.
When blood sugars are low, a molecule called glucoside locks NNC2215 in the ‘off’ position so it won’t lower blood sugars further. When blood sugar levels rise, glucose (sugar) in the blood replaces the glucoside, switching NNC2215 ‘on’ so it will bring blood sugars down.
In studies with rats and pigs, researchers found NNC2215’s switch worked as they’d hoped. It was able to turn on and off in response to changing blood sugar levels. And it was as effective as regular insulin at lowering high blood sugar, without causing it to drop too low.
NNC2215 hasn’t been tested in humans yet, and there will be many more steps before it could be. But these findings are encouraging.
Dr Elizabeth Robertson, our Director of Research, said:
“This research represents a significant step forward in the global effort to develop the next generation of ‘smart’ insulins. The hope is that these will ease the constant challenge of managing blood sugar highs and lows, and improve the physical and mental health of millions of people worldwide with diabetes who rely on insulin therapy. "We’re excited to part of this endeavour through our Type 1 Diabetes Grand Challenge programme .”
World-first stem cell transplant helps person with type 1 produce insulin
A new type of stem cell treatment, created from a person’s own cells, is showing early promise in allowing people with type 1 diabetes to make their own insulin.
Stem cells are like shape-shifters - they can turn into almost any type of cell the body needs. In the quest for new treatments and a cure for type 1 diabetes, scientists have been working to turn stem cells into insulin-making beta cells.
The hope is these stem cells-turned-beta cells could replace those that have been destroyed by the immune system in people with type 1 diabetes.
Until now, most clinical trials of stem cell therapies in people with type 1 diabetes have used donor stem cells. Because these stem cells are foreign, trial participants need to take immunosuppressant drugs to prevent their immune system from recognising and destroying them. These drugs come with significant side effects.
In a new study, published in Cell , a 25-year-old woman with type 1 diabetes became the first person in the world to receive a stem cell transplant created from her own cells. Scientists hope that this approach could reduce or eliminate the need for immunosuppressants.
Researchers at Peking University, Beijing, extracted cells from the participant and used chemicals in the lab to make what are known as chemically induced pluripotent stem cells. They then reprogrammed the stem cells to become beta cells, and injected around 1.5 million into the woman’s stomach.
Two-and-a-half months after this procedure, the woman’s new beta cells were producing enough insulin for her to stop insulin injections. A year later, she was still producing her own insulin, and her blood sugars were in range for 98% of the time. The researchers also didn’t see any major safety concerns.
This is an important and exciting development in stem cell therapies for type 1 diabetes. But the participant in this trial was already taking immunosuppressants because of a prior liver transplant. This means we can’t be sure if the new type of stem cell procedure alone was the reason she didn’t reject the transplanted cells, or if the immunosuppressants played a role.
So far, two other participants have also had the stem cell procedure, and the researchers are set to report their results soon. There will then need to be more studies, with more people to check if and how well the treatment works.
To speed up progress and get us to effective beta cell therapies quicker, we’re investing in cutting-edge research through the Type 1 Diabetes Grand Challenge .
Regenerating cells with electrical impulses in people with type 2 diabetes
Some people with type 2 diabetes were able to stop taking insulin after receiving a new procedure, which uses electrical impulses to improve the body’s response to insulin, alongside the blood sugar lowering medication semaglutide .
Researchers at the Amsterdam University Medical Centre ran the first trial of a new treatment, called Recellularisation via Electroporation Therapy (ReCET). It aims to improve the body’s sensitivity to its own insulin using pulsed electrical fields that target cells in the gut involved in blood sugar control.
The idea is to regenerate these cells, making them better at using insulin and bringing down blood sugar levels.
In the trial, 14 people living with type 2 diabetes had the ReCET procedure, which is done under sedation. They then began taking an existing type 2 diabetes medication: semaglutide, a GLP-1 agonist . This medication helps the body to produce more insulin.
Participants were followed up at six, 12 and 24 months. The study was set up to look at the safety of the ReCET procedure and promisingly there weren’t any serious safety concerns reported.
Over the two years, 12 of the 14 people (86%) no longer needed to take insulin to keep their blood sugars stayed in a target range.
This is exciting. But we need to keep in mind this was an early safety study, and the research wasn’t designed to look at the treatment’s effectiveness. As participants were taking a GLP-1 medication it also isn’t clear if the ReCET procedure gave any additional benefit.
Next, it will be important to compare ReCET with other existing type 2 treatments in head-to-head trials, including against GLP1-s alone.
We’ll also need to see if any benefits last longer term and which groups of people with type 2 it could hold potential to help. The team are already working on more studies, so answers will be coming.
Dr Celine Busch, lead author of the study, said:
“These findings are very encouraging, suggesting that ReCET is a safe and feasible procedure that, when combined with semaglutide, can effectively eliminate the need for insulin therapy.”
These results were presented at United European Gastroenterology Week (UEGW) 2024 in Vienna.
New driver of diabetic retinopathy discovered
With our funding, researchers at Queen’s University Belfast have uncovered a new factor that could help explain why diabetic retinopathy progresses and causes vision problems. The research shows a protein, called Pentraxin 3 (PTX3), might be driving harmful inflammation in the eyes of people with diabetes.
Diabetic retinopathy is a common complication of diabetes caused by high blood sugar levels damaging blood vessels in the eye. Inflammation can make this eye damage worse, but scientists haven’t been sure what causes it.
Recent evidence has shown that PTX3 is made in the eye, so our researchers led by Professor Reinhold Medina delved into its role in retinopathy for the first time. They compared two groups of mice with diabetes: one group who had PTX3 in the retina and a second group who didn’t make the protein. The researchers monitored the mice over nine months.
The researchers found that the mice without PTX3 showed less eye damage. These mice had fewer signs of inflammation in the retina and better vision, compared to the PTX3 mice. In the PTX3 mice, they saw that the protein activated certain cells that cause swelling.
The team then looked at samples from people with diabetic retinopathy and found that PTX3 levels were higher in people with more severe eye problems. These results suggest that PTX3 is involved in retinopathy progression by fuelling inflammation in the eye.
This knowledge opens the door to developing new treatments that target and block PTX3.
Dr Lucy Chambers, our Head of Research Communications, said:
“Eye problems are a frightening and too frequent complication of diabetes. By advancing our understanding of the biological factors contributing to eye damage, this research could take us closer to new and better treatments that help more people with diabetes avoid devastating harm to their sight.”
Share this Page

© The British Diabetic Association operating as Diabetes UK, a charity registered in England and Wales (no. 215199) and in Scotland (no. SC039136). A company limited by guarantee registered in England and Wales with (no.00339181) and registered office at Wells Lawrence House, 126 Back Church Lane London E1 1FH
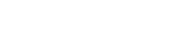
Towards Standardized Stem Cell Therapy in Type 2 Diabetes Mellitus: A Systematic Review
Affiliations.
- 1 Faculty of Medicine, Department of Histology, Universitas Indonesia, Jakarta, Indonesia.
- 2 Stem Cell and Tissue Engineering Research Center, Indonesian Medical Education and Research Institute (IMERI), Faculty of Medicine Universitas Indonesia, Jakarta, Indonesia.
- 3 Stem Cell Medical Technology Integrated Service Unit, Cipto Mangunkusumo Central Hospital - Faculty of Medicine Universitas Indonesia, Jakarta, Indonesia.
- 4 Tissue Engineering Program, Life Sciences Institute, National University of Singapore, Singapore.
- PMID: 29732994
- DOI: 10.2174/1574888X13666180502143657
Objective: To compile and analyze the published studies on cell therapy for type 2 diabetes mellitus (T2DM) to obtain a better insight into management of T2DM that involved stem cell therapy.
Methods: We searched all published studies in Pubmed/Medline, and Cochrane library, using keywords: ‘stem cell’ AND ‘therapy’ AND ‘diabetes type 2’. Inclusion criteria: original articles on the use of stem cells in humans with T2DM. Exclusion criteria: articles in the non-English literature, studies on T2DM complications that did not assess both adverse events and any of the common diabetes study outcomes. Data collection: type of study, number of cases, and all data that were related to outcome and adverse events. Data were analyzed descriptively to conclude the possible cause of adverse reactions, and which protocols gave a satisfactory outcome.
Results: We collected 25 original articles, out of which 17 studies did not have controls and were classified as case reports, while there were 8 studies that were controlled clinical trials. Most studies used autologous bone marrow mononuclear cells (BM-MNCs) or autologous or allogeneic mesenchymal stem cells (MSCs) from various sources. Adverse events were mild and mostly intervention related. Efficacy of autologous BM-MNCs that were given via interventional route was comparable to Wharton jelly or umbilical cord MSCs that were given via intravenous (IV), Intra muscular (IM), or subcutaneous (SC) route.
Conclusion: Further controlled studies that compare BM-MNCs to BM-MSCs or WJ-MSCs or UCSCs are recommended to prove their comparable efficacy. In addition, studies that compare various routes of administration (IV, IM or SC) versus the more invasive interventional routes are needed.
Keywords: Type 2 diabetes mellitus; Wharton’s jelly; bone marrow; mesenchymal stem cells; mononuclear cells; umbilical cord..
Copyright© Bentham Science Publishers; For any queries, please email at [email protected].
Publication types
- Systematic Review
- Bone Marrow Cells / cytology*
- Cell Differentiation / physiology
- Cell- and Tissue-Based Therapy* / methods
- Diabetes Mellitus, Type 2 / therapy*
- Mesenchymal Stem Cell Transplantation* / methods
- Mesenchymal Stem Cells / cytology*
An official website of the United States government
Official websites use .gov A .gov website belongs to an official government organization in the United States.
Secure .gov websites use HTTPS A lock ( Lock Locked padlock icon ) or https:// means you've safely connected to the .gov website. Share sensitive information only on official, secure websites.
- Publications
- Account settings
- Advanced Search
- Journal List

Stem cells: past, present, and future
Wojciech zakrzewski, maciej dobrzyński, maria szymonowicz, zbigniew rybak.
- Author information
- Article notes
- Copyright and License information
Corresponding author.
Collection date 2019.
Open Access This article is distributed under the terms of the Creative Commons Attribution 4.0 International License ( http://creativecommons.org/licenses/by/4.0/ ), which permits unrestricted use, distribution, and reproduction in any medium, provided you give appropriate credit to the original author(s) and the source, provide a link to the Creative Commons license, and indicate if changes were made. The Creative Commons Public Domain Dedication waiver ( http://creativecommons.org/publicdomain/zero/1.0/ ) applies to the data made available in this article, unless otherwise stated.
In recent years, stem cell therapy has become a very promising and advanced scientific research topic. The development of treatment methods has evoked great expectations. This paper is a review focused on the discovery of different stem cells and the potential therapies based on these cells. The genesis of stem cells is followed by laboratory steps of controlled stem cell culturing and derivation. Quality control and teratoma formation assays are important procedures in assessing the properties of the stem cells tested. Derivation methods and the utilization of culturing media are crucial to set proper environmental conditions for controlled differentiation. Among many types of stem tissue applications, the use of graphene scaffolds and the potential of extracellular vesicle-based therapies require attention due to their versatility. The review is summarized by challenges that stem cell therapy must overcome to be accepted worldwide. A wide variety of possibilities makes this cutting edge therapy a turning point in modern medicine, providing hope for untreatable diseases.
Keywords: Stem cells, Differentiation, Pluripotency, Induced pluripotent stem cell (iPSC), Teratoma formation assay, Stem cell derivation, Growth media, Tissue banks, Tissue transplantation
Stem cell classification
Stem cells are unspecialized cells of the human body. They are able to differentiate into any cell of an organism and have the ability of self-renewal. Stem cells exist both in embryos and adult cells. There are several steps of specialization. Developmental potency is reduced with each step, which means that a unipotent stem cell is not able to differentiate into as many types of cells as a pluripotent one. This chapter will focus on stem cell classification to make it easier for the reader to comprehend the following chapters.
Totipotent stem cells are able to divide and differentiate into cells of the whole organism. Totipotency has the highest differentiation potential and allows cells to form both embryo and extra-embryonic structures. One example of a totipotent cell is a zygote, which is formed after a sperm fertilizes an egg. These cells can later develop either into any of the three germ layers or form a placenta. After approximately 4 days, the blastocyst’s inner cell mass becomes pluripotent. This structure is the source of pluripotent cells.
Pluripotent stem cells (PSCs) form cells of all germ layers but not extraembryonic structures, such as the placenta. Embryonic stem cells (ESCs) are an example. ESCs are derived from the inner cell mass of preimplantation embryos. Another example is induced pluripotent stem cells (iPSCs) derived from the epiblast layer of implanted embryos. Their pluripotency is a continuum, starting from completely pluripotent cells such as ESCs and iPSCs and ending on representatives with less potency—multi-, oligo- or unipotent cells. One of the methods to assess their activity and spectrum is the teratoma formation assay. iPSCs are artificially generated from somatic cells, and they function similarly to PSCs. Their culturing and utilization are very promising for present and future regenerative medicine.
Multipotent stem cells have a narrower spectrum of differentiation than PSCs, but they can specialize in discrete cells of specific cell lineages. One example is a haematopoietic stem cell, which can develop into several types of blood cells. After differentiation, a haematopoietic stem cell becomes an oligopotent cell. Its differentiation abilities are then restricted to cells of its lineage. However, some multipotent cells are capable of conversion into unrelated cell types, which suggests naming them pluripotent cells.
Oligopotent stem cells can differentiate into several cell types. A myeloid stem cell is an example that can divide into white blood cells but not red blood cells.
Unipotent stem cells are characterized by the narrowest differentiation capabilities and a special property of dividing repeatedly. Their latter feature makes them a promising candidate for therapeutic use in regenerative medicine. These cells are only able to form one cell type, e.g. dermatocytes.
Stem cell biology
A blastocyst is formed after the fusion of sperm and ovum fertilization. Its inner wall is lined with short-lived stem cells, namely, embryonic stem cells. Blastocysts are composed of two distinct cell types: the inner cell mass (ICM), which develops into epiblasts and induces the development of a foetus, and the trophectoderm (TE). Blastocysts are responsible for the regulation of the ICM microenvironment. The TE continues to develop and forms the extraembryonic support structures needed for the successful origin of the embryo, such as the placenta. As the TE begins to form a specialized support structure, the ICM cells remain undifferentiated, fully pluripotent and proliferative [ 1 ]. The pluripotency of stem cells allows them to form any cell of the organism. Human embryonic stem cells (hESCs) are derived from the ICM. During the process of embryogenesis, cells form aggregations called germ layers: endoderm, mesoderm and ectoderm (Fig. 1 ), each eventually giving rise to differentiated cells and tissues of the foetus and, later on, the adult organism [ 2 ]. After hESCs differentiate into one of the germ layers, they become multipotent stem cells, whose potency is limited to only the cells of the germ layer. This process is short in human development. After that, pluripotent stem cells occur all over the organism as undifferentiated cells, and their key abilities are proliferation by the formation of the next generation of stem cells and differentiation into specialized cells under certain physiological conditions.
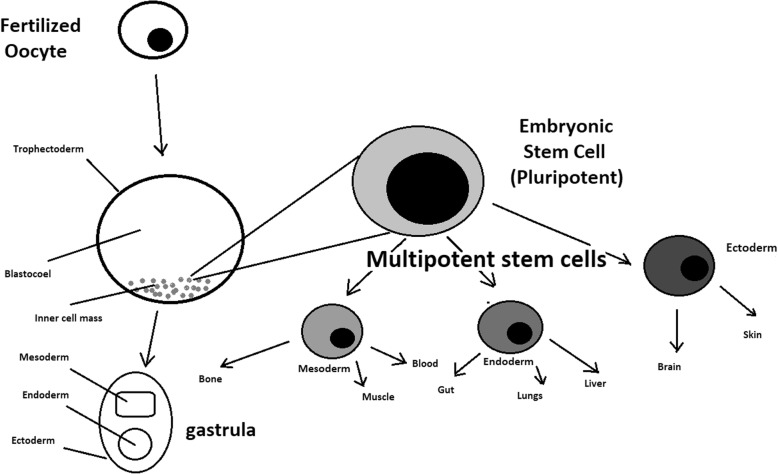
Oocyte development and formation of stem cells: the blastocoel, which is formed from oocytes, consists of embryonic stem cells that later differentiate into mesodermal, ectodermal, or endodermal cells. Blastocoel develops into the gastrula
Signals that influence the stem cell specialization process can be divided into external, such as physical contact between cells or chemical secretion by surrounding tissue, and internal, which are signals controlled by genes in DNA.
Stem cells also act as internal repair systems of the body. The replenishment and formation of new cells are unlimited as long as an organism is alive. Stem cell activity depends on the organ in which they are in; for example, in bone marrow, their division is constant, although in organs such as the pancreas, division only occurs under special physiological conditions.
Stem cell functional division
Whole-body development.
During division, the presence of different stem cells depends on organism development. Somatic stem cell ESCs can be distinguished. Although the derivation of ESCs without separation from the TE is possible, such a combination has growth limits. Because proliferating actions are limited, co-culture of these is usually avoided.
ESCs are derived from the inner cell mass of the blastocyst, which is a stage of pre-implantation embryo ca. 4 days after fertilization. After that, these cells are placed in a culture dish filled with culture medium. Passage is an inefficient but popular process of sub-culturing cells to other dishes. These cells can be described as pluripotent because they are able to eventually differentiate into every cell type in the organism. Since the beginning of their studies, there have been ethical restrictions connected to the medical use of ESCs in therapies. Most embryonic stem cells are developed from eggs that have been fertilized in an in vitro clinic, not from eggs fertilized in vivo.
Somatic or adult stem cells are undifferentiated and found among differentiated cells in the whole body after development. The function of these cells is to enable the healing, growth, and replacement of cells that are lost each day. These cells have a restricted range of differentiation options. Among many types, there are the following:
Mesenchymal stem cells are present in many tissues. In bone marrow, these cells differentiate mainly into the bone, cartilage, and fat cells. As stem cells, they are an exception because they act pluripotently and can specialize in the cells of any germ layer.
Neural cells give rise to nerve cells and their supporting cells—oligodendrocytes and astrocytes.
Haematopoietic stem cells form all kinds of blood cells: red, white, and platelets.
Skin stem cells form, for example, keratinocytes, which form a protective layer of skin.
The proliferation time of somatic stem cells is longer than that of ESCs. It is possible to reprogram adult stem cells back to their pluripotent state. This can be performed by transferring the adult nucleus into the cytoplasm of an oocyte or by fusion with the pluripotent cell. The same technique was used during cloning of the famous Dolly sheep.
hESCs are involved in whole-body development. They can differentiate into pluripotent, totipotent, multipotent, and unipotent cells (Fig. 2 ) [ 2 ].
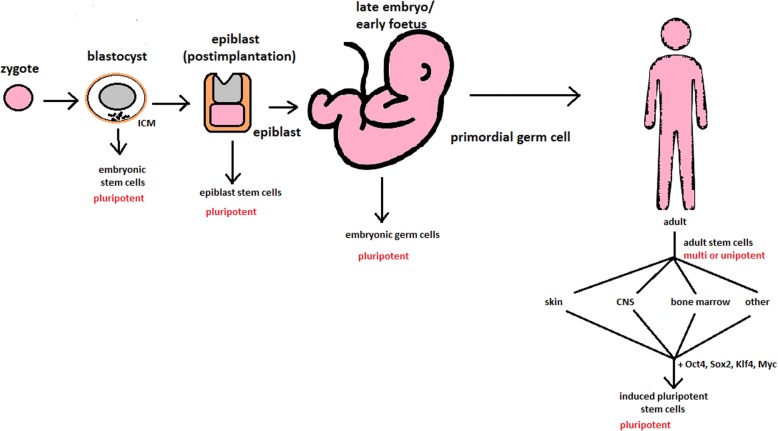
Changes in the potency of stem cells in human body development. Potency ranges from pluripotent cells of the blastocyst to unipotent cells of a specific tissue in a human body such as the skin, CNS, or bone marrow. Reversed pluripotency can be achieved by the formation of induced pluripotent stem cells using either octamer-binding transcription factor (Oct4), sex-determining region Y (Sox2), Kruppel-like factor 4 (Klf4), or the Myc gene
Pluripotent cells can be named totipotent if they can additionally form extraembryonic tissues of the embryo. Multipotent cells are restricted in differentiating to each cell type of given tissue. When tissue contains only one lineage of cells, stem cells that form them are called either called oligo- or unipotent.
iPSC quality control and recognition by morphological differences
The comparability of stem cell lines from different individuals is needed for iPSC lines to be used in therapeutics [ 3 ]. Among critical quality procedures, the following can be distinguished:
Short tandem repeat analysis—This is the comparison of specific loci on the DNA of the samples. It is used in measuring an exact number of repeating units. One unit consists of 2 to 13 nucleotides repeating many times on the DNA strand. A polymerase chain reaction is used to check the lengths of short tandem repeats. The genotyping procedure of source tissue, cells, and iPSC seed and master cell banks is recommended.
Identity analysis—The unintentional switching of lines, resulting in other stem cell line contamination, requires rigorous assay for cell line identification.
Residual vector testing—An appearance of reprogramming vectors integrated into the host genome is hazardous, and testing their presence is a mandatory procedure. It is a commonly used procedure for generating high-quality iPSC lines. An acceptable threshold in high-quality research-grade iPSC line collections is ≤ 1 plasmid copies per 100 cells. During the procedure, 2 different regions, common to all plasmids, should be used as specific targets, such as EBNA and CAG sequences [ 3 ]. To accurately represent the test reactions, a standard curve needs to be prepared in a carrier of gDNA from a well-characterized hPSC line. For calculations of plasmid copies per cell, it is crucial to incorporate internal reference gDNA sequences to allow the quantification of, for example, ribonuclease P (RNaseP) or human telomerase reverse transcriptase (hTERT).
Karyotype—A long-term culture of hESCs can accumulate culture-driven mutations [ 4 ]. Because of that, it is crucial to pay additional attention to genomic integrity. Karyotype tests can be performed by resuscitating representative aliquots and culturing them for 48–72 h before harvesting cells for karyotypic analysis. If abnormalities are found within the first 20 karyotypes, the analysis must be repeated on a fresh sample. When this situation is repeated, the line is evaluated as abnormal. Repeated abnormalities must be recorded. Although karyology is a crucial procedure in stem cell quality control, the single nucleotide polymorphism (SNP) array, discussed later, has approximately 50 times higher resolution.
Viral testing—When assessing the quality of stem cells, all tests for harmful human adventitious agents must be performed (e.g. hepatitis C or human immunodeficiency virus). This procedure must be performed in the case of non-xeno-free culture agents.
Bacteriology—Bacterial or fungal sterility tests can be divided into culture- or broth-based tests. All the procedures must be recommended by pharmacopoeia for the jurisdiction in which the work is performed.
Single nucleotide polymorphism arrays—This procedure is a type of DNA microarray that detects population polymorphisms by enabling the detection of subchromosomal changes and the copy-neutral loss of heterozygosity, as well as an indication of cellular transformation. The SNP assay consists of three components. The first is labelling fragmented nucleic acid sequences with fluorescent dyes. The second is an array that contains immobilized allele-specific oligonucleotide (ASO) probes. The last component detects, records, and eventually interprets the signal.
Flow cytometry—This is a technique that utilizes light to count and profile cells in a heterogeneous fluid mixture. It allows researchers to accurately and rapidly collect data from heterogeneous fluid mixtures with live cells. Cells are passed through a narrow channel one by one. During light illumination, sensors detect light emitted or refracted from the cells. The last step is data analysis, compilation and integration into a comprehensive picture of the sample.
Phenotypic pluripotency assays—Recognizing undifferentiated cells is crucial in successful stem cell therapy. Among other characteristics, stem cells appear to have a distinct morphology with a high nucleus to cytoplasm ratio and a prominent nucleolus. Cells appear to be flat with defined borders, in contrast to differentiating colonies, which appear as loosely located cells with rough borders [ 5 ]. It is important that images of ideal and poor quality colonies for each cell line are kept in laboratories, so whenever there is doubt about the quality of culture, it can always be checked according to the representative image. Embryoid body formation or directed differentiation of monolayer cultures to produce cell types representative of all three embryonic germ layers must be performed. It is important to note that colonies cultured under different conditions may have different morphologies [ 6 ].
Histone modification and DNA methylation—Quality control can be achieved by using epigenetic analysis tools such as histone modification or DNA methylation. When stem cells differentiate, the methylation process silences pluripotency genes, which reduces differentiation potential, although other genes may undergo demethylation to become expressed [ 7 ]. It is important to emphasize that stem cell identity, together with its morphological characteristics, is also related to its epigenetic profile [ 8 , 9 ]. According to Brindley [ 10 ], there is a relationship between epigenetic changes, pluripotency, and cell expansion conditions, which emphasizes that unmethylated regions appear to be serum-dependent.
hESC derivation and media
hESCs can be derived using a variety of methods, from classic culturing to laser-assisted methodologies or microsurgery [ 11 ]. hESC differentiation must be specified to avoid teratoma formation (see Fig. 3 ).
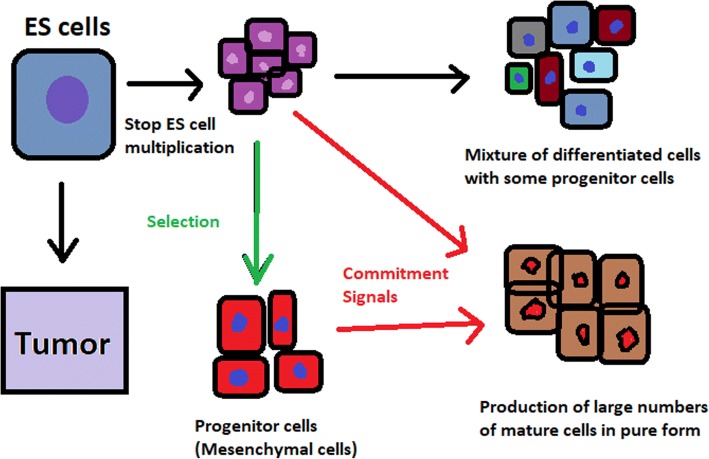
Spontaneous differentiation of hESCs causes the formation of a heterogeneous cell population. There is a different result, however, when commitment signals (in forms of soluble factors and culture conditions) are applied and enable the selection of progenitor cells
hESCs spontaneously differentiate into embryonic bodies (EBs) [ 12 ]. EBs can be studied instead of embryos or animals to predict their effects on early human development. There are many different methods for acquiring EBs, such as bioreactor culture [ 13 ], hanging drop culture [ 12 ], or microwell technology [ 14 , 15 ]. These methods allow specific precursors to form in vitro [ 16 ].
The essential part of these culturing procedures is a separation of inner cell mass to culture future hESCs (Fig. 4 ) [ 17 ]. Rosowski et al. [ 18 ] emphasizes that particular attention must be taken in controlling spontaneous differentiation. When the colony reaches the appropriate size, cells must be separated. The occurrence of pluripotent cells lasts for 1–2 days. Because the classical utilization of hESCs caused ethical concerns about gastrulas used during procedures, Chung et al. [ 19 ] found out that it is also possible to obtain hESCs from four cell embryos, leaving a higher probability of embryo survival. Additionally, Zhang et al. [ 20 ] used only in vitro fertilization growth-arrested cells.
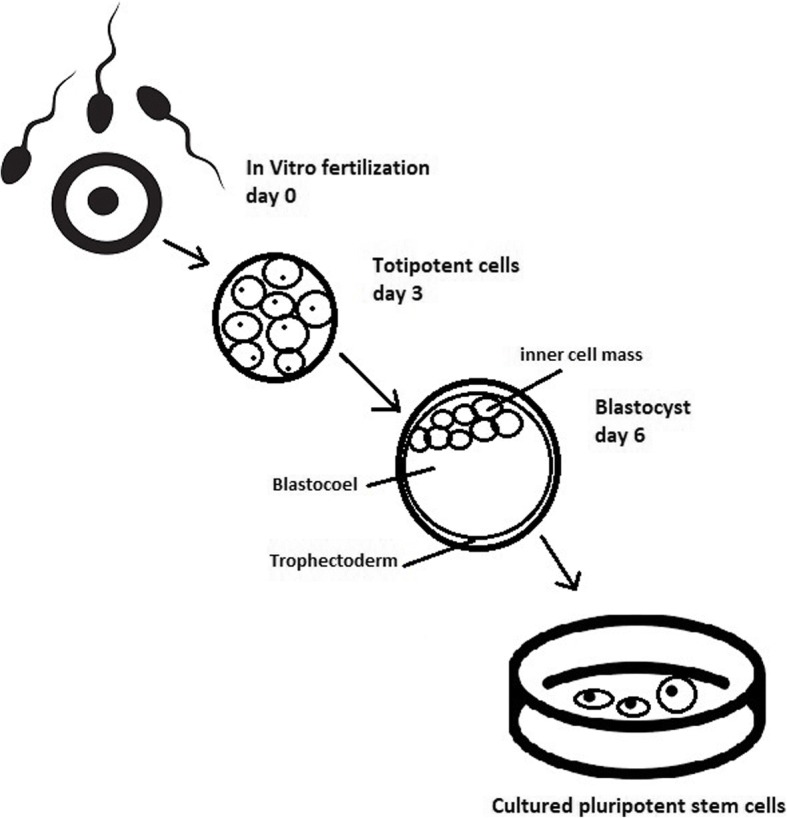
Culturing of pluripotent stem cells in vitro. Three days after fertilization, totipotent cells are formed. Blastocysts with ICM are formed on the sixth day after fertilization. Pluripotent stem cells from ICM can then be successfully transmitted on a dish
Cell passaging is used to form smaller clusters of cells on a new culture surface [ 21 ]. There are four important passaging procedures.
Enzymatic dissociation is a cutting action of enzymes on proteins and adhesion domains that bind the colony. It is a gentler method than the manual passage. It is crucial to not leave hESCs alone after passaging. Solitary cells are more sensitive and can easily undergo cell death; collagenase type IV is an example [ 22 , 23 ].
Manual passage , on the other hand, focuses on using cell scratchers. The selection of certain cells is not necessary. This should be done in the early stages of cell line derivation [ 24 ].
Trypsin utilization allows a healthy, automated hESC passage. Good Manufacturing Practice (GMP)-grade recombinant trypsin is widely available in this procedure [ 24 ]. However, there is a risk of decreasing the pluripotency and viability of stem cells [ 25 ]. Trypsin utilization can be halted with an inhibitor of the protein rho-associated protein kinase (ROCK) [ 26 ].
Ethylenediaminetetraacetic acid ( EDTA ) indirectly suppresses cell-to-cell connections by chelating divalent cations. Their suppression promotes cell dissociation [ 27 ].
Stem cells require a mixture of growth factors and nutrients to differentiate and develop. The medium should be changed each day.
Traditional culture methods used for hESCs are mouse embryonic fibroblasts (MEFs) as a feeder layer and bovine serum [ 28 ] as a medium. Martin et al. [ 29 ] demonstrated that hESCs cultured in the presence of animal products express the non-human sialic acid, N -glycolylneuraminic acid (NeuGc). Feeder layers prevent uncontrolled proliferation with factors such as leukaemia inhibitory factor (LIF) [ 30 ].
First feeder layer-free culture can be supplemented with serum replacement, combined with laminin [ 31 ]. This causes stable karyotypes of stem cells and pluripotency lasting for over a year.
Initial culturing media can be serum (e.g. foetal calf serum FCS), artificial replacement such as synthetic serum substitute (SSS), knockout serum replacement (KOSR), or StemPro [ 32 ]. The simplest culture medium contains only eight essential elements: DMEM/F12 medium, selenium, NaHCO 3, l -ascorbic acid, transferrin, insulin, TGFβ1, and FGF2 [ 33 ]. It is not yet fully known whether culture systems developed for hESCs can be allowed without adaptation in iPSC cultures.
Turning point in stem cell therapy
The turning point in stem cell therapy appeared in 2006, when scientists Shinya Yamanaka, together with Kazutoshi Takahashi, discovered that it is possible to reprogram multipotent adult stem cells to the pluripotent state. This process avoided endangering the foetus’ life in the process. Retrovirus-mediated transduction of mouse fibroblasts with four transcription factors (Oct-3/4, Sox2, KLF4, and c-Myc) [ 34 ] that are mainly expressed in embryonic stem cells could induce the fibroblasts to become pluripotent (Fig. 5 ) [ 35 ]. This new form of stem cells was named iPSCs. One year later, the experiment also succeeded with human cells [ 36 ]. After this success, the method opened a new field in stem cell research with a generation of iPSC lines that can be customized and biocompatible with the patient. Recently, studies have focused on reducing carcinogenesis and improving the conduction system.
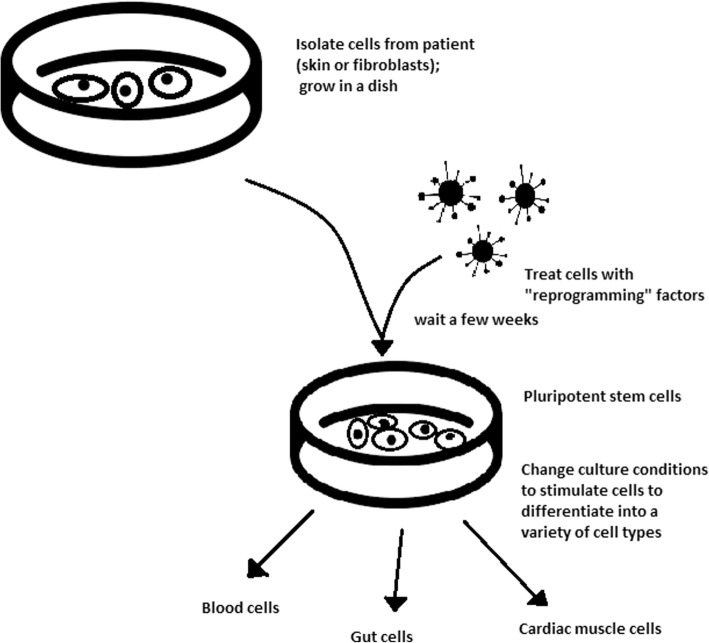
Retroviral-mediated transduction induces pluripotency in isolated patient somatic cells. Target cells lose their role as somatic cells and, once again, become pluripotent and can differentiate into any cell type of human body
The turning point was influenced by former discoveries that happened in 1962 and 1987.
The former discovery was about scientist John Gurdon successfully cloning frogs by transferring a nucleus from a frog’s somatic cells into an oocyte. This caused a complete reversion of somatic cell development [ 37 ]. The results of his experiment became an immense discovery since it was previously believed that cell differentiation is a one-way street only, but his experiment suggested the opposite and demonstrated that it is even possible for a somatic cell to again acquire pluripotency [ 38 ].
The latter was a discovery made by Davis R.L. that focused on fibroblast DNA subtraction. Three genes were found that originally appeared in myoblasts. The enforced expression of only one of the genes, named myogenic differentiation 1 (Myod1), caused the conversion of fibroblasts into myoblasts, showing that reprogramming cells is possible, and it can even be used to transform cells from one lineage to another [ 39 ].
Although pluripotency can occur naturally only in embryonic stem cells, it is possible to induce terminally differentiated cells to become pluripotent again. The process of direct reprogramming converts differentiated somatic cells into iPSC lines that can form all cell types of an organism. Reprogramming focuses on the expression of oncogenes such as Myc and Klf4 (Kruppel-like factor 4). This process is enhanced by a downregulation of genes promoting genome stability, such as p53. Additionally, cell reprogramming involves histone alteration. All these processes can cause potential mutagenic risk and later lead to an increased number of mutations. Quinlan et al. [ 40 ] checked fully pluripotent mouse iPSCs using whole genome DNA sequencing and structural variation (SV) detection algorithms. Based on those studies, it was confirmed that although there were single mutations in the non-genetic region, there were non-retrotransposon insertions. This led to the conclusion that current reprogramming methods can produce fully pluripotent iPSCs without severe genomic alterations.
During the course of development from pluripotent hESCs to differentiated somatic cells, crucial changes appear in the epigenetic structure of these cells. There is a restriction or permission of the transcription of genes relevant to each cell type. When somatic cells are being reprogrammed using transcription factors, all the epigenetic architecture has to be reconditioned to achieve iPSCs with pluripotency [ 41 ]. However, cells of each tissue undergo specific somatic genomic methylation. This influences transcription, which can further cause alterations in induced pluripotency [ 42 ].
Source of iPSCs
Because pluripotent cells can propagate indefinitely and differentiate into any kind of cell, they can be an unlimited source, either for replacing lost or diseased tissues. iPSCs bypass the need for embryos in stem cell therapy. Because they are made from the patient’s own cells, they are autologous and no longer generate any risk of immune rejection.
At first, fibroblasts were used as a source of iPSCs. Because a biopsy was needed to achieve these types of cells, the technique underwent further research. Researchers investigated whether more accessible cells could be used in the method. Further, other cells were used in the process: peripheral blood cells, keratinocytes, and renal epithelial cells found in urine. An alternative strategy to stem cell transplantation can be stimulating a patient’s endogenous stem cells to divide or differentiate, occurring naturally when skin wounds are healing. In 2008, pancreatic exocrine cells were shown to be reprogrammed to functional, insulin-producing beta cells [ 43 ].
The best stem cell source appears to be the fibroblasts, which is more tempting in the case of logistics since its stimulation can be fast and better controlled [ 44 ].
Teratoma formation assay
The self-renewal and differentiation capabilities of iPSCs have gained significant interest and attention in regenerative medicine sciences. To study their abilities, a quality-control assay is needed, of which one of the most important is the teratoma formation assay. Teratomas are benign tumours. Teratomas are capable of rapid growth in vivo and are characteristic because of their ability to develop into tissues of all three germ layers simultaneously. Because of the high pluripotency of teratomas, this formation assay is considered an assessment of iPSC’s abilities [ 45 ].
Teratoma formation rate, for instance, was observed to be elevated in human iPSCs compared to that in hESCs [ 46 ]. This difference may be connected to different differentiation methods and cell origins. Most commonly, the teratoma assay involves an injection of examined iPSCs subcutaneously or under the testis or kidney capsule in mice, which are immune-deficient [ 47 ]. After injection, an immature but recognizable tissue can be observed, such as the kidney tubules, bone, cartilage, or neuroepithelium [ 30 ]. The injection site may have an impact on the efficiency of teratoma formation [ 48 ].
There are three groups of markers used in this assay to differentiate the cells of germ layers. For endodermal tissue, there is insulin/C-peptide and alpha-1 antitrypsin [ 49 ]. For the mesoderm, derivatives can be used, e.g. cartilage matrix protein for the bone and alcian blue for the cartilage. As ectodermal markers, class III B botulin or keratin can be used for keratinocytes.
Teratoma formation assays are considered the gold standard for demonstrating the pluripotency of human iPSCs, demonstrating their possibilities under physiological conditions. Due to their actual tissue formation, they could be used for the characterization of many cell lineages [ 50 ].
Directed differentiation
To be useful in therapy, stem cells must be converted into desired cell types as necessary or else the whole regenerative medicine process will be pointless. Differentiation of ESCs is crucial because undifferentiated ESCs can cause teratoma formation in vivo. Understanding and using signalling pathways for differentiation is an important method in successful regenerative medicine. In directed differentiation, it is likely to mimic signals that are received by cells when they undergo successive stages of development [ 51 ]. The extracellular microenvironment plays a significant role in controlling cell behaviour. By manipulating the culture conditions, it is possible to restrict specific differentiation pathways and generate cultures that are enriched in certain precursors in vitro. However, achieving a similar effect in vivo is challenging. It is crucial to develop culture conditions that will allow the promotion of homogenous and enhanced differentiation of ESCs into functional and desired tissues.
Regarding the self-renewal of embryonic stem cells, Hwang et al. [ 52 ] noted that the ideal culture method for hESC-based cell and tissue therapy would be a defined culture free of either the feeder layer or animal components. This is because cell and tissue therapy requires the maintenance of large quantities of undifferentiated hESCs, which does not make feeder cells suitable for such tasks.
Most directed differentiation protocols are formed to mimic the development of an inner cell mass during gastrulation. During this process, pluripotent stem cells differentiate into ectodermal, mesodermal, or endodermal progenitors. Mall molecules or growth factors induce the conversion of stem cells into appropriate progenitor cells, which will later give rise to the desired cell type. There is a variety of signal intensities and molecular families that may affect the establishment of germ layers in vivo, such as fibroblast growth factors (FGFs) [ 53 ]; the Wnt family [ 54 ] or superfamily of transforming growth factors—β(TGFβ); and bone morphogenic proteins (BMP) [ 55 ]. Each candidate factor must be tested on various concentrations and additionally applied to various durations because the precise concentrations and times during which developing cells in embryos are influenced during differentiation are unknown. For instance, molecular antagonists of endogenous BMP and Wnt signalling can be used for ESC formation of ectoderm [ 56 ]. However, transient Wnt and lower concentrations of the TGFβ family trigger mesodermal differentiation [ 57 ]. Regarding endoderm formation, a higher activin A concentration may be required [ 58 , 59 ].
There are numerous protocols about the methods of forming progenitors of cells of each of germ layers, such as cardiomyocytes [ 60 ], hepatocytes [ 61 ], renal cells [ 62 ], lung cells [ 63 , 64 ], motor neurons [ 65 ], intestinal cells [ 66 ], or chondrocytes [ 67 ].
Directed differentiation of either iPSCs or ESCs into, e.g. hepatocytes, could influence and develop the study of the molecular mechanisms in human liver development. In addition, it could also provide the possibility to form exogenous hepatocytes for drug toxicity testing [ 68 ].
Levels of concentration and duration of action with a specific signalling molecule can cause a variety of factors. Unfortunately, for now, a high cost of recombinant factors is likely to limit their use on a larger scale in medicine. The more promising technique focuses on the use of small molecules. These can be used for either activating or deactivating specific signalling pathways. They enhance reprogramming efficiency by creating cells that are compatible with the desired type of tissue. It is a cheaper and non-immunogenic method.
One of the successful examples of small-molecule cell therapies is antagonists and agonists of the Hedgehog pathway. They show to be very useful in motor neuron regeneration [ 69 ]. Endogenous small molecules with their function in embryonic development can also be used in in vitro methods to induce the differentiation of cells; for example, retinoic acid, which is responsible for patterning the nervous system in vivo [ 70 ], surprisingly induced retinal cell formation when the laboratory procedure involved hESCs [ 71 ].
The efficacy of differentiation factors depends on functional maturity, efficiency, and, finally, introducing produced cells to their in vivo equivalent. Topography, shear stress, and substrate rigidity are factors influencing the phenotype of future cells [ 72 ].
The control of biophysical and biochemical signals, the biophysical environment, and a proper guide of hESC differentiation are important factors in appropriately cultured stem cells.
Stem cell utilization and their manufacturing standards and culture systems
The European Medicines Agency and the Food and Drug Administration have set Good Manufacturing Practice (GMP) guidelines for safe and appropriate stem cell transplantation. In the past, protocols used for stem cell transplantation required animal-derived products [ 73 ].
The risk of introducing animal antigens or pathogens caused a restriction in their use. Due to such limitations, the technique required an obvious update [ 74 ]. Now, it is essential to use xeno-free equivalents when establishing cell lines that are derived from fresh embryos and cultured from human feeder cell lines [ 75 ]. In this method, it is crucial to replace any non-human materials with xeno-free equivalents [ 76 ].
NutriStem with LN-511, TeSR2 with human recombinant laminin (LN-511), and RegES with human foreskin fibroblasts (HFFs) are commonly used xeno-free culture systems [ 33 ]. There are many organizations and international initiatives, such as the National Stem Cell Bank, that provide stem cell lines for treatment or medical research [ 77 ].
Stem cell use in medicine
Stem cells have great potential to become one of the most important aspects of medicine. In addition to the fact that they play a large role in developing restorative medicine, their study reveals much information about the complex events that happen during human development.
The difference between a stem cell and a differentiated cell is reflected in the cells’ DNA. In the former cell, DNA is arranged loosely with working genes. When signals enter the cell and the differentiation process begins, genes that are no longer needed are shut down, but genes required for the specialized function will remain active. This process can be reversed, and it is known that such pluripotency can be achieved by interaction in gene sequences. Takahashi and Yamanaka [ 78 ] and Loh et al. [ 79 ] discovered that octamer-binding transcription factor 3 and 4 (Oct3/4), sex determining region Y (SRY)-box 2 and Nanog genes function as core transcription factors in maintaining pluripotency. Among them, Oct3/4 and Sox2 are essential for the generation of iPSCs.
Many serious medical conditions, such as birth defects or cancer, are caused by improper differentiation or cell division. Currently, several stem cell therapies are possible, among which are treatments for spinal cord injury, heart failure [ 80 ], retinal and macular degeneration [ 81 ], tendon ruptures, and diabetes type 1 [ 82 ]. Stem cell research can further help in better understanding stem cell physiology. This may result in finding new ways of treating currently incurable diseases.
Haematopoietic stem cell transplantation
Haematopoietic stem cells are important because they are by far the most thoroughly characterized tissue-specific stem cell; after all, they have been experimentally studied for more than 50 years. These stem cells appear to provide an accurate paradigm model system to study tissue-specific stem cells, and they have potential in regenerative medicine.
Multipotent haematopoietic stem cell (HSC) transplantation is currently the most popular stem cell therapy. Target cells are usually derived from the bone marrow, peripheral blood, or umbilical cord blood [ 83 ]. The procedure can be autologous (when the patient’s own cells are used), allogenic (when the stem cell comes from a donor), or syngeneic (from an identical twin). HSCs are responsible for the generation of all functional haematopoietic lineages in blood, including erythrocytes, leukocytes, and platelets. HSC transplantation solves problems that are caused by inappropriate functioning of the haematopoietic system, which includes diseases such as leukaemia and anaemia. However, when conventional sources of HSC are taken into consideration, there are some important limitations. First, there is a limited number of transplantable cells, and an efficient way of gathering them has not yet been found. There is also a problem with finding a fitting antigen-matched donor for transplantation, and viral contamination or any immunoreactions also cause a reduction in efficiency in conventional HSC transplantations. Haematopoietic transplantation should be reserved for patients with life-threatening diseases because it has a multifactorial character and can be a dangerous procedure. iPSC use is crucial in this procedure. The use of a patient’s own unspecialized somatic cells as stem cells provides the greatest immunological compatibility and significantly increases the success of the procedure.
Stem cells as a target for pharmacological testing
Stem cells can be used in new drug tests. Each experiment on living tissue can be performed safely on specific differentiated cells from pluripotent cells. If any undesirable effect appears, drug formulas can be changed until they reach a sufficient level of effectiveness. The drug can enter the pharmacological market without harming any live testers. However, to test the drugs properly, the conditions must be equal when comparing the effects of two drugs. To achieve this goal, researchers need to gain full control of the differentiation process to generate pure populations of differentiated cells.
Stem cells as an alternative for arthroplasty
One of the biggest fears of professional sportsmen is getting an injury, which most often signifies the end of their professional career. This applies especially to tendon injuries, which, due to current treatment options focusing either on conservative or surgical treatment, often do not provide acceptable outcomes. Problems with the tendons start with their regeneration capabilities. Instead of functionally regenerating after an injury, tendons merely heal by forming scar tissues that lack the functionality of healthy tissues. Factors that may cause this failed healing response include hypervascularization, deposition of calcific materials, pain, or swelling [ 84 ].
Additionally, in addition to problems with tendons, there is a high probability of acquiring a pathological condition of joints called osteoarthritis (OA) [ 85 ]. OA is common due to the avascular nature of articular cartilage and its low regenerative capabilities [ 86 ]. Although arthroplasty is currently a common procedure in treating OA, it is not ideal for younger patients because they can outlive the implant and will require several surgical procedures in the future. These are situations where stem cell therapy can help by stopping the onset of OA [ 87 ]. However, these procedures are not well developed, and the long-term maintenance of hyaline cartilage requires further research.
Osteonecrosis of the femoral hip (ONFH) is a refractory disease associated with the collapse of the femoral head and risk of hip arthroplasty in younger populations [ 88 ]. Although total hip arthroplasty (THA) is clinically successful, it is not ideal for young patients, mostly due to the limited lifetime of the prosthesis. An increasing number of clinical studies have evaluated the therapeutic effect of stem cells on ONFH. Most of the authors demonstrated positive outcomes, with reduced pain, improved function, or avoidance of THA [ 89 – 91 ].
Rejuvenation by cell programming
Ageing is a reversible epigenetic process. The first cell rejuvenation study was published in 2011 [ 92 ]. Cells from aged individuals have different transcriptional signatures, high levels of oxidative stress, dysfunctional mitochondria, and shorter telomeres than in young cells [ 93 ]. There is a hypothesis that when human or mouse adult somatic cells are reprogrammed to iPSCs, their epigenetic age is virtually reset to zero [ 94 ]. This was based on an epigenetic model, which explains that at the time of fertilization, all marks of parenteral ageing are erased from the zygote’s genome and its ageing clock is reset to zero [ 95 ].
In their study, Ocampo et al. [ 96 ] used Oct4, Sox2, Klf4, and C-myc genes (OSKM genes) and affected pancreas and skeletal muscle cells, which have poor regenerative capacity. Their procedure revealed that these genes can also be used for effective regenerative treatment [ 97 ]. The main challenge of their method was the need to employ an approach that does not use transgenic animals and does not require an indefinitely long application. The first clinical approach would be preventive, focused on stopping or slowing the ageing rate. Later, progressive rejuvenation of old individuals can be attempted. In the future, this method may raise some ethical issues, such as overpopulation, leading to lower availability of food and energy.
For now, it is important to learn how to implement cell reprogramming technology in non-transgenic elder animals and humans to erase marks of ageing without removing the epigenetic marks of cell identity.
Cell-based therapies
Stem cells can be induced to become a specific cell type that is required to repair damaged or destroyed tissues (Fig. 6 ). Currently, when the need for transplantable tissues and organs outweighs the possible supply, stem cells appear to be a perfect solution for the problem. The most common conditions that benefit from such therapy are macular degenerations [ 98 ], strokes [ 99 ], osteoarthritis [ 89 , 90 ], neurodegenerative diseases, and diabetes [ 100 ]. Due to this technique, it can become possible to generate healthy heart muscle cells and later transplant them to patients with heart disease.
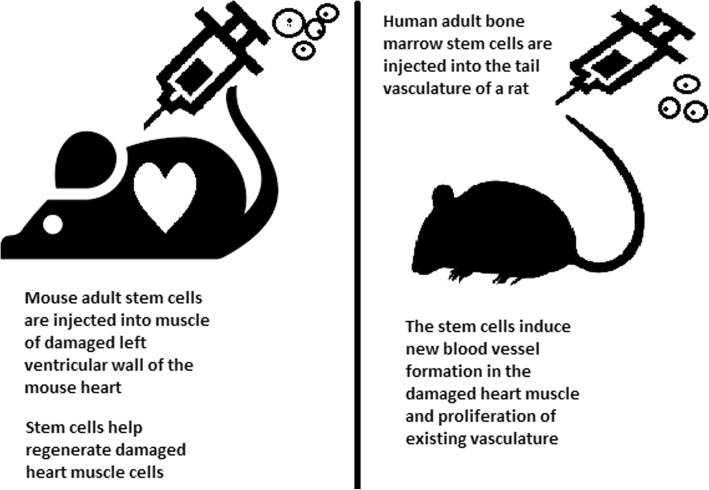
Stem cell experiments on animals. These experiments are one of the many procedures that proved stem cells to be a crucial factor in future regenerative medicine
In the case of type 1 diabetes, insulin-producing cells in the pancreas are destroyed due to an autoimmunological reaction. As an alternative to transplantation therapy, it can be possible to induce stem cells to differentiate into insulin-producing cells [ 101 ].
Stem cells and tissue banks
iPS cells with their theoretically unlimited propagation and differentiation abilities are attractive for the present and future sciences. They can be stored in a tissue bank to be an essential source of human tissue used for medical examination. The problem with conventional differentiated tissue cells held in the laboratory is that their propagation features diminish after time. This does not occur in iPSCs.
The umbilical cord is known to be rich in mesenchymal stem cells. Due to its cryopreservation immediately after birth, its stem cells can be successfully stored and used in therapies to prevent the future life-threatening diseases of a given patient.
Stem cells of human exfoliated deciduous teeth (SHED) found in exfoliated deciduous teeth has the ability to develop into more types of body tissues than other stem cells [ 102 ] (Table 1 ). Techniques of their collection, isolation, and storage are simple and non-invasive. Among the advantages of banking, SHED cells are:
Guaranteed donor-match autologous transplant that causes no immune reaction and rejection of cells [ 103 ]
Simple and painless for both child and parent
Less than one third of the cost of cord blood storage
Not subject to the same ethical concerns as embryonic stem cells [ 104 ]
In contrast to cord blood stem cells, SHED cells are able to regenerate into solid tissues such as connective, neural, dental, or bone tissue [ 105 , 106 ]
SHED can be useful for close relatives of the donor
Types of stem cells in human exfoliated deciduous teeth
Fertility diseases
In 2011, two researchers, Katsuhiko Hayashi et al. [ 107 ], showed in an experiment on mice that it is possible to form sperm from iPSCs. They succeeded in delivering healthy and fertile pups in infertile mice. The experiment was also successful for female mice, where iPSCs formed fully functional eggs .
Young adults at risk of losing their spermatogonial stem cells (SSC), mostly cancer patients, are the main target group that can benefit from testicular tissue cryopreservation and autotransplantation. Effective freezing methods for adult and pre-pubertal testicular tissue are available [ 108 ].
Qiuwan et al. [ 109 ] provided important evidence that human amniotic epithelial cell (hAEC) transplantation could effectively improve ovarian function by inhibiting cell apoptosis and reducing inflammation in injured ovarian tissue of mice, and it could be a promising strategy for the management of premature ovarian failure or insufficiency in female cancer survivors.
For now, reaching successful infertility treatments in humans appears to be only a matter of time, but there are several challenges to overcome. First, the process needs to have high efficiency; second, the chances of forming tumours instead of eggs or sperm must be maximally reduced. The last barrier is how to mature human sperm and eggs in the lab without transplanting them to in vivo conditions, which could cause either a tumour risk or an invasive procedure.
Therapy for incurable neurodegenerative diseases
Thanks to stem cell therapy, it is possible not only to delay the progression of incurable neurodegenerative diseases such as Parkinson’s disease, Alzheimer’s disease (AD), and Huntington disease, but also, most importantly, to remove the source of the problem. In neuroscience, the discovery of neural stem cells (NSCs) has nullified the previous idea that adult CNS were not capable of neurogenesis [ 110 , 111 ]. Neural stem cells are capable of improving cognitive function in preclinical rodent models of AD [ 112 – 114 ]. Awe et al. [ 115 ] clinically derived relevant human iPSCs from skin punch biopsies to develop a neural stem cell-based approach for treating AD. Neuronal degeneration in Parkinson’s disease (PD) is focal, and dopaminergic neurons can be efficiently generated from hESCs. PD is an ideal disease for iPSC-based cell therapy [ 116 ]. However, this therapy is still in an experimental phase ( https://www.ncbi.nlm.nih.gov/pmc/articles/PMC4539501 /). Brain tissue from aborted foetuses was used on patients with Parkinson’s disease [ 117 ]. Although the results were not uniform, they showed that therapies with pure stem cells are an important and achievable therapy.
Stem cell use in dentistry
Teeth represent a very challenging material for regenerative medicine. They are difficult to recreate because of their function in aspects such as articulation, mastication, or aesthetics due to their complicated structure. Currently, there is a chance for stem cells to become more widely used than synthetic materials. Teeth have a large advantage of being the most natural and non-invasive source of stem cells.
For now, without the use of stem cells, the most common periodontological treatments are either growth factors, grafts, or surgery. For example, there are stem cells in periodontal ligament [ 118 , 119 ], which are capable of differentiating into osteoblasts or cementoblasts, and their functions were also assessed in neural cells [ 120 ]. Tissue engineering is a successful method for treating periodontal diseases. Stem cells of the root apical areas are able to recreate periodontal ligament. One of the possible methods of tissue engineering in periodontology is gene therapy performed using adenoviruses-containing growth factors [ 121 ].
As a result of animal studies, dentin regeneration is an effective process that results in the formation of dentin bridges [ 122 ].
Enamel is more difficult to regenerate than dentin. After the differentiation of ameloblastoma cells into the enamel, the former is destroyed, and reparation is impossible. Medical studies have succeeded in differentiating bone marrow stem cells into ameloblastoma [ 123 ].
Healthy dental tissue has a high amount of regular stem cells, although this number is reduced when tissue is either traumatized or inflamed [ 124 ]. There are several dental stem cell groups that can be isolated (Fig. 7 ).
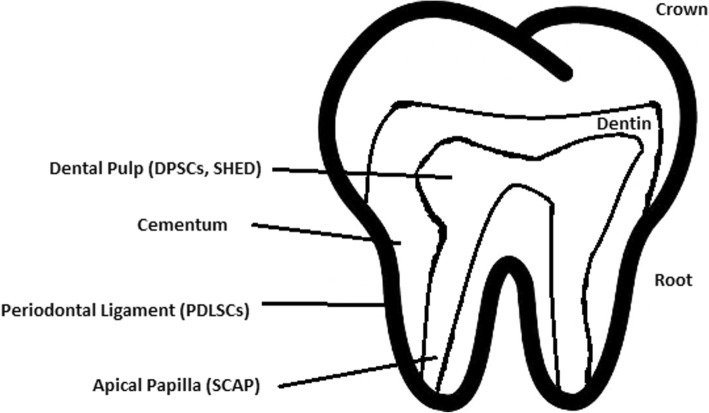
Localization of stem cells in dental tissues. Dental pulp stem cells (DPSCs) and human deciduous teeth stem cells (SHED) are located in the dental pulp. Periodontal ligaments stem cells are located in the periodontal ligament. Apical papilla consists of stem cells from the apical papilla (SCAP)
Dental pulp stem cell (DPSC)
These were the first dental stem cells isolated from the human dental pulp, which were [ 125 ] located inside dental pulp (Table 2 ). They have osteogenic and chondrogenic potential. Mesenchymal stem cells (MSCs) of the dental pulp, when isolated, appear highly clonogenic; they can be isolated from adult tissue (e.g. bone marrow, adipose tissue) and foetal (e.g. umbilical cord) [ 126 ] tissue, and they are able to differentiate densely [ 127 ]. MSCs differentiate into odontoblast-like cells and osteoblasts to form dentin and bone. Their best source locations are the third molars [ 125 ]. DPSCs are the most useful dental source of tissue engineering due to their easy surgical accessibility, cryopreservation possibility, increased production of dentin tissues compared to non-dental stem cells, and their anti-inflammatory abilities. These cells have the potential to be a source for maxillofacial and orthopaedic reconstructions or reconstructions even beyond the oral cavity. DPSCs are able to generate all structures of the developed tooth [ 128 ]. In particular, beneficial results in the use of DPSCs may be achieved when combined with other new therapies, such as periodontal tissue photobiomodulation (laser stimulation), which is an efficient technique in the stimulation of proliferation and differentiation into distinct cell types [ 129 ]. DPSCs can be induced to form neural cells to help treat neurological deficits.
Detailed information about the differentiation of DPSCs and the studies connected to them [ 176 ]
Stem cells of human exfoliated deciduous teeth (SHED) have a faster rate of proliferation than DPSCs and differentiate into an even greater number of cells, e.g. other mesenchymal and non-mesenchymal stem cell derivatives, such as neural cells [ 130 ]. These cells possess one major disadvantage: they form a non-complete dentin/pulp-like complex in vivo. SHED do not undergo the same ethical concerns as embryonic stem cells. Both DPSCs and SHED are able to form bone-like tissues in vivo [ 131 ] and can be used for periodontal, dentin, or pulp regeneration. DPSCs and SHED can be used in treating, for example, neural deficits [ 132 ]. DPSCs alone were tested and successfully applied for alveolar bone and mandible reconstruction [ 133 ].
Periodontal ligament stem cells (PDLSCs)
These cells are used in periodontal ligament or cementum tissue regeneration. They can differentiate into mesenchymal cell lineages to produce collagen-forming cells, adipocytes, cementum tissue, Sharpey’s fibres, and osteoblast-like cells in vitro. PDLSCs exist both on the root and alveolar bone surfaces; however, on the latter, these cells have better differentiation abilities than on the former [ 134 ]. PDLSCs have become the first treatment for periodontal regeneration therapy because of their safety and efficiency [ 135 , 136 ].
Stem cells from apical papilla (SCAP)
These cells are mesenchymal structures located within immature roots. They are isolated from human immature permanent apical papilla. SCAP are the source of odontoblasts and cause apexogenesis. These stem cells can be induced in vitro to form odontoblast-like cells, neuron-like cells, or adipocytes. SCAP have a higher capacity of proliferation than DPSCs, which makes them a better choice for tissue regeneration [ 137 , 138 ].
Dental follicle stem cells (DFCs)
These cells are loose connective tissues surrounding the developing tooth germ. DFCs contain cells that can differentiate into cementoblasts, osteoblasts, and periodontal ligament cells [ 139 , 140 ]. Additionally, these cells proliferate after even more than 30 passages [ 141 ]. DFCs are most commonly extracted from the sac of a third molar. When DFCs are combined with a treated dentin matrix, they can form a root-like tissue with a pulp-dentin complex and eventually form tooth roots [ 141 ]. When DFC sheets are induced by Hertwig’s epithelial root sheath cells, they can produce periodontal tissue; thus, DFCs represent a very promising material for tooth regeneration [ 142 ].
Pulp regeneration in endodontics
Dental pulp stem cells can differentiate into odontoblasts. There are few methods that enable the regeneration of the pulp.
The first is an ex vivo method. Proper stem cells are grown on a scaffold before they are implanted into the root channel [ 143 ].
The second is an in vivo method. This method focuses on injecting stem cells into disinfected root channels after the opening of the in vivo apex. Additionally, the use of a scaffold is necessary to prevent the movement of cells towards other tissues. For now, only pulp-like structures have been created successfully.
Methods of placing stem cells into the root channel constitute are either soft scaffolding [ 144 ] or the application of stem cells in apexogenesis or apexification. Immature teeth are the best source [ 145 ]. Nerve and blood vessel network regeneration are extremely vital to keep pulp tissue healthy.
The potential of dental stem cells is mainly regarding the regeneration of damaged dentin and pulp or the repair of any perforations; in the future, it appears to be even possible to generate the whole tooth. Such an immense success would lead to the gradual replacement of implant treatments. Mandibulary and maxillary defects can be one of the most complicated dental problems for stem cells to address.
Acquiring non-dental tissue cells by dental stem cell differentiation
In 2013, it was reported that it is possible to grow teeth from stem cells obtained extra-orally, e.g. from urine [ 146 ]. Pluripotent stem cells derived from human urine were induced and generated tooth-like structures. The physical properties of the structures were similar to natural ones except for hardness [ 127 ]. Nonetheless, it appears to be a very promising technique because it is non-invasive and relatively low-cost, and somatic cells can be used instead of embryonic cells. More importantly, stem cells derived from urine did not form any tumours, and the use of autologous cells reduces the chances of rejection [ 147 ].
Use of graphene in stem cell therapy
Over recent years, graphene and its derivatives have been increasingly used as scaffold materials to mediate stem cell growth and differentiation [ 148 ]. Both graphene and graphene oxide (GO) represent high in-plane stiffness [ 149 ]. Because graphene has carbon and aromatic network, it works either covalently or non-covalently with biomolecules; in addition to its superior mechanical properties, graphene offers versatile chemistry. Graphene exhibits biocompatibility with cells and their proper adhesion. It also tested positively for enhancing the proliferation or differentiation of stem cells [ 148 ]. After positive experiments, graphene revealed great potential as a scaffold and guide for specific lineages of stem cell differentiation [ 150 ]. Graphene has been successfully used in the transplantation of hMSCs and their guided differentiation to specific cells. The acceleration skills of graphene differentiation and division were also investigated. It was discovered that graphene can serve as a platform with increased adhesion for both growth factors and differentiation chemicals. It was also discovered that π-π binding was responsible for increased adhesion and played a crucial role in inducing hMSC differentiation [ 150 ].
Therapeutic potential of extracellular vesicle-based therapies
Extracellular vesicles (EVs) can be released by virtually every cell of an organism, including stem cells [ 151 ], and are involved in intercellular communication through the delivery of their mRNAs, lipids, and proteins. As Oh et al. [ 152 ] prove, stem cells, together with their paracrine factors—exosomes—can become potential therapeutics in the treatment of, e.g. skin ageing. Exosomes are small membrane vesicles secreted by most cells (30–120 nm in diameter) [ 153 ]. When endosomes fuse with the plasma membrane, they become exosomes that have messenger RNAs (mRNAs) and microRNAs (miRNAs), some classes of non-coding RNAs (IncRNAs) and several proteins that originate from the host cell [ 154 ]. IncRNAs can bind to specific loci and create epigenetic regulators, which leads to the formation of epigenetic modifications in recipient cells. Because of this feature, exosomes are believed to be implicated in cell-to-cell communication and the progression of diseases such as cancer [ 155 ]. Recently, many studies have also shown the therapeutic use of exosomes derived from stem cells, e.g. skin damage and renal or lung injuries [ 156 ].
In skin ageing, the most important factor is exposure to UV light, called “photoageing” [ 157 ], which causes extrinsic skin damage, characterized by dryness, roughness, irregular pigmentation, lesions, and skin cancers. In intrinsic skin ageing, on the other hand, the loss of elasticity is a characteristic feature. The skin dermis consists of fibroblasts, which are responsible for the synthesis of crucial skin elements, such as procollagen or elastic fibres. These elements form either basic framework extracellular matrix constituents of the skin dermis or play a major role in tissue elasticity. Fibroblast efficiency and abundance decrease with ageing [ 158 ]. Stem cells can promote the proliferation of dermal fibroblasts by secreting cytokines such as platelet-derived growth factor (PDGF), transforming growth factor β (TGF-β), and basic fibroblast growth factor. Huh et al. [ 159 ] mentioned that a medium of human amniotic fluid-derived stem cells (hAFSC) positively affected skin regeneration after longwave UV-induced (UVA, 315–400 nm) photoageing by increasing the proliferation and migration of dermal fibroblasts. It was discovered that, in addition to the induction of fibroblast physiology, hAFSC transplantation also improved diseases in cases of renal pathology, various cancers, or stroke [ 160 , 161 ].
Oh [ 162 ] also presented another option for the treatment of skin wounds, either caused by physical damage or due to diabetic ulcers. Induced pluripotent stem cell-conditioned medium (iPSC-CM) without any animal-derived components induced dermal fibroblast proliferation and migration.
Natural cutaneous wound healing is divided into three steps: haemostasis/inflammation, proliferation, and remodelling. During the crucial step of proliferation, fibroblasts migrate and increase in number, indicating that it is a critical step in skin repair, and factors such as iPSC-CM that impact it can improve the whole cutaneous wound healing process. Paracrine actions performed by iPSCs are also important for this therapeutic effect [ 163 ]. These actions result in the secretion of cytokines such as TGF-β, interleukin (IL)-6, IL-8, monocyte chemotactic protein-1 (MCP-1), vascular endothelial growth factor (VEGF), platelet-derived growth factor-AA (PDGF-AA), and basic fibroblast growth factor (bFGF). Bae et al. [ 164 ] mentioned that TGF-β induced the migration of keratinocytes. It was also demonstrated that iPSC factors can enhance skin wound healing in vivo and in vitro when Zhou et al. [ 165 ] enhanced wound healing, even after carbon dioxide laser resurfacing in an in vivo study.
Peng et al. [ 166 ] investigated the effects of EVs derived from hESCs on in vitro cultured retinal glial, progenitor Müller cells, which are known to differentiate into retinal neurons. EVs appear heterogeneous in size and can be internalized by cultured Müller cells, and their proteins are involved in the induction and maintenance of stem cell pluripotency. These stem cell-derived vesicles were responsible for the neuronal trans-differentiation of cultured Müller cells exposed to them. However, the research article points out that the procedure was accomplished only on in vitro acquired retina.
Challenges concerning stem cell therapy
Although stem cells appear to be an ideal solution for medicine, there are still many obstacles that need to be overcome in the future. One of the first problems is ethical concern.
The most common pluripotent stem cells are ESCs. Therapies concerning their use at the beginning were, and still are, the source of ethical conflicts. The reason behind it started when, in 1998, scientists discovered the possibility of removing ESCs from human embryos. Stem cell therapy appeared to be very effective in treating many, even previously incurable, diseases. The problem was that when scientists isolated ESCs in the lab, the embryo, which had potential for becoming a human, was destroyed (Fig. 8 ). Because of this, scientists, seeing a large potential in this treatment method, focused their efforts on making it possible to isolate stem cells without endangering their source—the embryo.
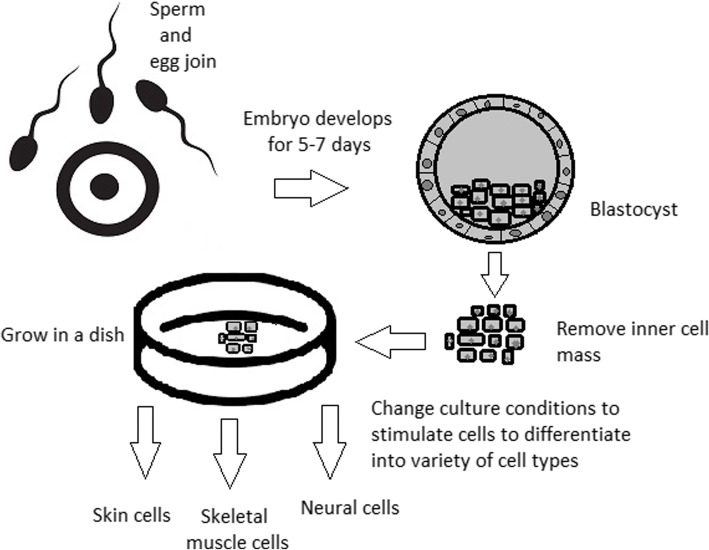
Use of inner cell mass pluripotent stem cells and their stimulation to differentiate into desired cell types
For now, while hESCs still remain an ethically debatable source of cells, they are potentially powerful tools to be used for therapeutic applications of tissue regeneration. Because of the complexity of stem cell control systems, there is still much to be learned through observations in vitro. For stem cells to become a popular and widely accessible procedure, tumour risk must be assessed. The second problem is to achieve successful immunological tolerance between stem cells and the patient’s body. For now, one of the best ideas is to use the patient’s own cells and devolve them into their pluripotent stage of development.
New cells need to have the ability to fully replace lost or malfunctioning natural cells. Additionally, there is a concern about the possibility of obtaining stem cells without the risk of morbidity or pain for either the patient or the donor. Uncontrolled proliferation and differentiation of cells after implementation must also be assessed before its use in a wide variety of regenerative procedures on living patients [ 167 ].
One of the arguments that limit the use of iPSCs is their infamous role in tumourigenicity. There is a risk that the expression of oncogenes may increase when cells are being reprogrammed. In 2008, a technique was discovered that allowed scientists to remove oncogenes after a cell achieved pluripotency, although it is not efficient yet and takes a longer amount of time. The process of reprogramming may be enhanced by deletion of the tumour suppressor gene p53, but this gene also acts as a key regulator of cancer, which makes it impossible to remove in order to avoid more mutations in the reprogrammed cell. The low efficiency of the process is another problem, which is progressively becoming reduced with each year. At first, the rate of somatic cell reprogramming in Yamanaka’s study was up to 0.1%. The use of transcription factors creates a risk of genomic insertion and further mutation of the target cell genome. For now, the only ethically acceptable operation is an injection of hESCs into mouse embryos in the case of pluripotency evaluation [ 168 ].
Stem cell obstacles in the future
Pioneering scientific and medical advances always have to be carefully policed in order to make sure they are both ethical and safe. Because stem cell therapy already has a large impact on many aspects of life, it should not be treated differently.
Currently, there are several challenges concerning stem cells. First, the most important one is about fully understanding the mechanism by which stem cells function first in animal models. This step cannot be avoided. For the widespread, global acceptance of the procedure, fear of the unknown is the greatest challenge to overcome.
The efficiency of stem cell-directed differentiation must be improved to make stem cells more reliable and trustworthy for a regular patient. The scale of the procedure is another challenge. Future stem cell therapies may be a significant obstacle. Transplanting new, fully functional organs made by stem cell therapy would require the creation of millions of working and biologically accurate cooperating cells. Bringing such complicated procedures into general, widespread regenerative medicine will require interdisciplinary and international collaboration.
The identification and proper isolation of stem cells from a patient’s tissues is another challenge. Immunological rejection is a major barrier to successful stem cell transplantation. With certain types of stem cells and procedures, the immune system may recognize transplanted cells as foreign bodies, triggering an immune reaction resulting in transplant or cell rejection.
One of the ideas that can make stem cells a “failsafe” is about implementing a self-destruct option if they become dangerous. Further development and versatility of stem cells may cause reduction of treatment costs for people suffering from currently incurable diseases. When facing certain organ failure, instead of undergoing extraordinarily expensive drug treatment, the patient would be able to utilize stem cell therapy. The effect of a successful operation would be immediate, and the patient would avoid chronic pharmacological treatment and its inevitable side effects.
Although these challenges facing stem cell science can be overwhelming, the field is making great advances each day. Stem cell therapy is already available for treating several diseases and conditions. Their impact on future medicine appears to be significant.
After several decades of experiments, stem cell therapy is becoming a magnificent game changer for medicine. With each experiment, the capabilities of stem cells are growing, although there are still many obstacles to overcome. Regardless, the influence of stem cells in regenerative medicine and transplantology is immense. Currently, untreatable neurodegenerative diseases have the possibility of becoming treatable with stem cell therapy. Induced pluripotency enables the use of a patient’s own cells. Tissue banks are becoming increasingly popular, as they gather cells that are the source of regenerative medicine in a struggle against present and future diseases. With stem cell therapy and all its regenerative benefits, we are better able to prolong human life than at any time in history.
Acknowledgements
Not applicable.
This work is supported by Wrocław Medical University in Poland.
Availability of data and materials
Please contact author for data requests.
Abbreviations
Basic fibroblast growth factor
Bone morphogenic proteins
Dental follicle stem cells
Dental pulp stem cells
Embryonic bodies
Embryonic stem cells
Fibroblast growth factors
Good Manufacturing Practice
Graphene oxide
Human amniotic fluid-derived stem cells
Human embryonic stem cells
Human foreskin fibroblasts
Inner cell mass
Non-coding RNA
Induced pluripotent stem cells
In vitro fertilization
Knockout serum replacement
Leukaemia inhibitory factor
Monocyte chemotactic protein-1
Fibroblasts
Messenger RNA
Mesenchymal stem cells of dental pulp
Myogenic differentiation
Osteoarthritis
Octamer-binding transcription factor 3 and 4
Platelet-derived growth factor
Platelet-derived growth factor-AA
Periodontal ligament stem cells
Rho-associated protein kinase
Stem cells from apical papilla
Stem cells of human exfoliated deciduous teeth
Synthetic Serum Substitute
Trophectoderm
Vascular endothelial growth factor
Transforming growth factors
Authors’ contributions
WZ is the principal author and was responsible for the first draft of the manuscript. WZ and ZR were responsible for the concept of the review. MS, MD, and ZR were responsible for revising the article and for data acquisition. All authors read and approved the final manuscript.
Ethics approval and consent to participate
Consent for publication, competing interests.
The authors declare that they have no competing interests.
Publisher’s Note
Springer Nature remains neutral with regard to jurisdictional claims in published maps and institutional affiliations.
Contributor Information
Wojciech Zakrzewski, Email: [email protected].
Maciej Dobrzyński, Email: [email protected].
Maria Szymonowicz, Email: [email protected].
Zbigniew Rybak, Email: [email protected].
- 1. Sukoyan MA, Vatolin SY, et al. Embryonic stem cells derived from morulae, inner cell mass, and blastocysts of mink: comparisons of their pluripotencies. Embryo Dev. 1993;36(2):148–58 [ DOI ] [ PubMed ]
- 2. Larijani B, Esfahani EN, Amini P, Nikbin B, Alimoghaddam K, Amiri S, Malekzadeh R, Yazdi NM, Ghodsi M, Dowlati Y, Sahraian MA, Ghavamzadeh A. Stem cell therapy in treatment of different diseases. Acta Medica Iranica. 2012:79–96 https://www.ncbi.nlm.nih.gov/pubmed/22359076 . [ PubMed ]
- 3. Sullivan S, Stacey GN, Akazawa C, et al. Quality guidelines for clinical-grade human induced pluripotent stem cell lines. Regenerative Med. 2018; 10.2217/rme-2018-0095. [ DOI ] [ PubMed ]
- 4. Amps K, Andrews PW, et al. Screening ethnically diverse human embryonic stem cells identifies a chromosome 20 minimal amplicon conferring growth advantage. Nat. Biotechnol. 2011;29(12):1121–1144. doi: 10.1038/nbt.2051. [ DOI ] [ PMC free article ] [ PubMed ] [ Google Scholar ]
- 5. Amit M, Itskovitz-Eldor J. Atlas of human pluripotent stem cells: derivation and culturing. New York: Humana Press; 2012. [ Google Scholar ]
- 6. Ludwig TE, Bergendahl V, Levenstein ME, Yu J, Probasco MD, Thomson JA. Feeder-independent culture of human embryonic stem cells. Nat Methods. 2006;3:637–646. doi: 10.1038/nmeth902. [ DOI ] [ PubMed ] [ Google Scholar ]
- 7. Kang MI. Transitional CpG methylation between promoters and retroelements of tissue-specific genes during human mesenchymal cell differentiation. J. Cell Biochem. 2007;102:224–239. doi: 10.1002/jcb.21291. [ DOI ] [ PubMed ] [ Google Scholar ]
- 8. Vaes B, Craeye D, Pinxteren J. Quality control during manufacture of a stem cell therapeutic. BioProcess Int. 2012;10:50–5.
- 9. Bloushtain-Qimron N. Epigenetic patterns of embryonic and adult stem cells. Cell Cycle. 2009;8:809–817. doi: 10.4161/cc.8.6.7938. [ DOI ] [ PubMed ] [ Google Scholar ]
- 10. Brindley DA. Peak serum: implications of serum supply for cell therapy manufacturing. Regenerative Medicine. 2012;7:809–817. doi: 10.2217/rme.11.112. [ DOI ] [ PubMed ] [ Google Scholar ]
- 11. Solter D, Knowles BB. Immunosurgery of mouse blastocyst. Proc Natl Acad Sci U S A. 1975;72:5099–5102. doi: 10.1073/pnas.72.12.5099. [ DOI ] [ PMC free article ] [ PubMed ] [ Google Scholar ]
- 12. Hoepfl G, Gassmann M, Desbaillets I. Differentiating embryonic stem cells into embryoid bodies. Methods Mole Biol. 2004;254:79–98. doi: 10.1385/1-59259-741-6:079. [ DOI ] [ PubMed ] [ Google Scholar ]
- 13. Lim WF, Inoue-Yokoo T, Tan KS, Lai MI, Sugiyama D. Hematopoietic cell differentiation from embryonic and induced pluripotent stem cells. Stem Cell Res Ther. 2013;4(3):71. doi: 10.1186/scrt222. [ DOI ] [ PMC free article ] [ PubMed ] [ Google Scholar ]
- 14. Mohr JC, de Pablo JJ, Palecek SP. 3-D microwell culture of human embryonic stem cells. Biomaterials. 2006;27(36):6032–6042. doi: 10.1016/j.biomaterials.2006.07.012. [ DOI ] [ PubMed ] [ Google Scholar ]
- 15. Doetschman TC, Eistetter H, Katz M, Schmidt W, Kemler R. The in vitro development of blastocyst-derived embryonic stem cell lines: formation of the visceral yolk sac, blood islands, and myocardium. J Embryol Exp Morphol. 1985;87:27–45. [ PubMed ] [ Google Scholar ]
- 16. Kurosawa HY. Methods for inducing embryoid body formation: in vitro differentiation system of embryonic stem cells. J Biosci Bioeng. 2007;103:389–98. [ DOI ] [ PubMed ]
- 17. Heins N, Englund MC, Sjoblom C, Dahl U, Tonning A, Bergh C, Lindahl A, Hanson C, Semb H. Derivation, characterization, and differentiation of human embryonic stem cells. Stem Cells. 2004;22:367–76. [ DOI ] [ PubMed ]
- 18. Rosowski KA, Mertz AF, Norcross S, Dufresne ER, Horsley V. Edges of human embryonic stem cell colonies display distinct mechanical properties and differentiation potential. Sci Rep. 2015;5:Article number:14218. doi: 10.1038/srep14218. [ DOI ] [ PMC free article ] [ PubMed ] [ Google Scholar ]
- 19. Chung Y, Klimanskaya I, Becker S, Li T, Maserati M, Lu SJ, Zdravkovic T, Ilic D, Genbacev O, Fisher S, Krtolica A, Lanza R. Human embryonic stem cell lines generated without embryo destruction. Cell Stem Cell. 2008;2:113–117. doi: 10.1016/j.stem.2007.12.013. [ DOI ] [ PubMed ] [ Google Scholar ]
- 20. Zhang X, Stojkovic P, Przyborski S, Cooke M, Armstrong L, Lako M, Stojkovic M. Derivation of human embryonic stem cells from developing and arrested embryos. Stem Cells. 2006;24:2669–2676. doi: 10.1634/stemcells.2006-0377. [ DOI ] [ PubMed ] [ Google Scholar ]
- 21. Beers J, Gulbranson DR, George N, Siniscalchi LI, Jones J, Thomson JA, Chen G. Passaging and colony expansion of human pluripotent stem cells by enzyme-free dissociation in chemically defined culture conditions. Nat Protoc. 2012;7:2029–2040. doi: 10.1038/nprot.2012.130. [ DOI ] [ PMC free article ] [ PubMed ] [ Google Scholar ]
- 22. Ellerström C, Hyllner J, Strehl R. single cell enzymatic dissociation of human embryonic stem cells: a straightforward, robust, and standardized culture method. In: Turksen K, editor. Human embryonic stem cell protocols. Methods in molecular biology: Humana Press; 2009. p. 584. [ DOI ] [ PubMed ]
- 23. Heng BC, Liu H, Ge Z, Cao T. Mechanical dissociation of human embryonic stem cell colonies by manual scraping after collagenase treatment is much more detrimental to cellular viability than is trypsinization with gentle pipetting. Biotechnol Appl Biochem. 2010;47(1):33–37. doi: 10.1042/BA20060151. [ DOI ] [ PubMed ] [ Google Scholar ]
- 24. Ellerstrom C, Strehl R, Noaksson K, Hyllner J, Semb H. Facilitated expansion of human embryonic stem cells by single-cell enzymatic dissociation. Stem Cells. 2007;25:1690–1696. doi: 10.1634/stemcells.2006-0607. [ DOI ] [ PubMed ] [ Google Scholar ]
- 25. Brimble SN, Zeng X, Weiler DA, Luo Y, Liu Y, Lyons IG, Freed WJ, Robins AJ, Rao MS, Schulz TC. Karyotypic stability, genotyping, differentiation, feeder-free maintenance, and gene expression sampling in three human embryonic stem cell lines deri. Stem Cells Dev. 2004;13:585–597. doi: 10.1089/scd.2004.13.585. [ DOI ] [ PubMed ] [ Google Scholar ]
- 26. Watanabe K, Ueno M, Kamiya D, Nishiyama A, Matsumura M, Wataya T, Takahashi JB, Nishikawa S, Nishikawa S, Muguruma K, Sasai Y. A ROCK inhibitor permits survival of dissociated human embryonic stem cells. Nat Biotechnol. 2007;25:681–686. doi: 10.1038/nbt1310. [ DOI ] [ PubMed ] [ Google Scholar ]
- 27. Nie Y, Walsh P, Clarke DL, Rowley JA, Fellner T. Scalable passaging of adherent human pluripotent stem cells. 2014. 10.1371/journal.pone.0088012. [ DOI ] [ PMC free article ] [ PubMed ]
- 28. Thomson JA, Itskovitz-Eldor J, Shapiro SS, Waknitz MA, Swiergiel JJ, Marshall VS, Jones JM. Embryonic stem cell lines derived from human blastocysts. Science. 1998;282:1145–1147. doi: 10.1126/science.282.5391.1145. [ DOI ] [ PubMed ] [ Google Scholar ]
- 29. Martin MJ, Muotri A, Gage F, Varki A. Human embryonic stem cellsexpress an immunogenic nonhuman sialic acid. Nat. Med. 2005;11:228–232. doi: 10.1038/nm1181. [ DOI ] [ PubMed ] [ Google Scholar ]
- 30. Smith AG, Heath JK, Donaldson DD, Wong GG, Moreau J, Stahl M, Rogers D. Inhibition of pluripotential embryonic stem cell differentiation by purified polypeptides. Nature. 1988;336(6200):688–690. doi: 10.1038/336688a0. [ DOI ] [ PubMed ] [ Google Scholar ]
- 31. Xu C, Inokuma MS, Denham J, Golds K, Kundu P, Gold JD, Carpenter MK. Feeder-free growth of undifferentiated human embryonic stem cells. Nature Biotechnol. 2001;19:971–974. doi: 10.1038/nbt1001-971. [ DOI ] [ PubMed ] [ Google Scholar ]
- 32. Weathersbee PS, Pool TB, Ord T. Synthetic serum substitute (SSS): a globulin-enriched protein supplement for human embryo culture. J. Assist Reprod Genet. 1995;12:354–360. doi: 10.1007/BF02215726. [ DOI ] [ PubMed ] [ Google Scholar ]
- 33. Chen G, Gulbranson DR, Hou Z, Bolin JM, Ruotti V, Probasco MD, Smuga-Otto K, Howden SE, Diol NR, Propson NE, Wagner R, Lee GO, Antosiewicz-Bourget J, Teng JM, Thomson JA. Chemically defined conditions for human iPSC derivation and culture. Nat. Methods. 2011;8:424–429. doi: 10.1038/nmeth.1593. [ DOI ] [ PMC free article ] [ PubMed ] [ Google Scholar ]
- 34. Sommer CA, Mostoslavsky G. Experimental approaches for the generation of induced pluripotent stem cells. Stem Cell Res Ther. 2010;1:26. doi: 10.1186/scrt26. [ DOI ] [ PMC free article ] [ PubMed ] [ Google Scholar ]
- 35. Takahashi K, Yamanaka S. Induced pluripotent stem cells in medicine and biology. Development. 2013;140(12):2457–2461. doi: 10.1242/dev.092551. [ DOI ] [ PubMed ] [ Google Scholar ]
- 36. Shi D, Lu F, Wei Y, et al. Buffalos (Bubalus bubalis) cloned by nuclear transfer of somatic cells. Biol. Reprod. 2007;77:285–291. doi: 10.1095/biolreprod.107.060210. [ DOI ] [ PubMed ] [ Google Scholar ]
- 37. Gurdon JB. The developmental capacity of nuclei taken from intestinal epithelium cells of feeding tadpoles. Development. 1962;10:622–640. [ PubMed ] [ Google Scholar ]
- 38. Kain K. The birth of cloning: an interview with John Gurdon. Dis Model Mech. 2009;2(1–2):9–10. doi: 10.1242/dmm.002014. [ DOI ] [ PMC free article ] [ PubMed ] [ Google Scholar ]
- 39. Davis RL, Weintraub H, Lassar AB. Expression of a single transfected cDNA converts fibroblasts to myoblasts. Cell. 1987;24(51(6)):987–1000. doi: 10.1016/0092-8674(87)90585-x. [ DOI ] [ PubMed ] [ Google Scholar ]
- 40. Quinlan AR, Boland MJ, Leibowitz ML, et al. Genome sequencing of mouse induced pluripotent stem cells reveals retroelement stability and infrequent DNA rearrangement during reprogramming. Cell Stem Cell. 2011;9(4):366–373. doi: 10.1016/j.stem.2011.07.018. [ DOI ] [ PMC free article ] [ PubMed ] [ Google Scholar ]
- 41. Maherali N, Sridharan R, Xie W, Utika LJ, Eminli S, Arnold K, Stadtfeld M, Yachechko R, Tchieu J, Jaenisch R, Plath K, Hochedlinger K. Directly reprogrammed fibroblasts show global epigenetic remodeling and widespread tissue contribution. Cell Stem Cell. 2007;1:55–70. doi: 10.1016/j.stem.2007.05.014. [ DOI ] [ PubMed ] [ Google Scholar ]
- 42. Ohi Y, Qin H, Hong C, Blouin L, Polo JM, Guo T, Qi Z, Downey SL, Manos PD, Rossi DJ, Yu J, Hebrok M, Hochedlinger K, Costello JF, Song JS, Ramalho-Santos M. Incomplete DNA methylation underlines a transcriptional memory of somatic cells in human IPS cells. Nat Cell Biol. 2011;13:541–549. doi: 10.1038/ncb2239. [ DOI ] [ PMC free article ] [ PubMed ] [ Google Scholar ]
- 43. Zhou Q, Brown J, Kanarek A, Rajagopal J, Melton DA. In vivo reprogramming of adult pancreatic exocrine cells to beta-cells. Nature. 2008;455:627–632. doi: 10.1038/nature07314. [ DOI ] [ PMC free article ] [ PubMed ] [ Google Scholar ]
- 44. Hilfiker A, Kasper C, Hass R, Haverich A. Mesenchymal stem cells and progenitor cells in connective tissue engineering and regenerative medicine: is there a future for transplantation? Langenbecks Arch Surg. 2011;396:489–497. doi: 10.1007/s00423-011-0762-2. [ DOI ] [ PubMed ] [ Google Scholar ]
- 45. Zhang Wendy, Y., de Almeida Patricia, E., and Wu Joseph, C. Teratoma formation: a tool for monitoring pluripotency in stem cell research. StemBook, ed. The Stem Cell Research Community . June 12, 2012. 10.3824/stembook.1.53.1.
- 46. Narsinh KH, Sun N, Sanchez-Freire V, et al. Single cell transcriptional profiling reveals heterogeneity of human induced pluripotent stem cells. J Clin Invest. 2011;121(3):1217–1221. doi: 10.1172/JCI44635. [ DOI ] [ PMC free article ] [ PubMed ] [ Google Scholar ]
- 47. Gertow K, Przyborski S, Loring JF, Auerbach JM, Epifano O, Otonkoski T, Damjanov I, AhrlundRichter L. Isolation of human embryonic stem cell-derived teratomas for the assessment of pluripotency. Curr Protoc Stem Cell Biol . 2007, Chapter 1, Unit 1B 4. 3: 1B.4.1-1B.4.29. [ DOI ] [ PubMed ]
- 48. Cooke MJ, Stojkovic M, Przyborski SA. Growth of teratomas derived from human pluripotent stem cells is influenced by the graft site. Stem Cells Dev. 2006;15(2):254–259. doi: 10.1089/scd.2006.15.254. [ DOI ] [ PubMed ] [ Google Scholar ]
- 49. Przyborski SA. Differentiation of human embryonic stem cells after transplantation in immune-deficient mice. Stem Cells. 2005;23:1242–1250. doi: 10.1634/stemcells.2005-0014. [ DOI ] [ PubMed ] [ Google Scholar ]
- 50. Tannenbaum SE, Turetsky TT, Singer O, Aizenman E, Kirshberg S, Ilouz N, Gil Y, Berman-Zaken Y, Perlman TS, Geva N, Levy O, Arbell D, Simon A, Ben-Meir A, Shufaro Y, Laufer N, Reubinoff BE. Derivation of xeno-free and GMP-grade human embryonic stem cells- platforms for future clinical applications. PLoS One. 2012;7:e35325. doi: 10.1371/journal.pone.0035325. [ DOI ] [ PMC free article ] [ PubMed ] [ Google Scholar ]
- 51. Cohen DE, Melton D. Turning straw into gold: directing cell fate for regenerative medicine. Nat Rev Genet. 2011;12:243–252. doi: 10.1038/nrg2938. [ DOI ] [ PubMed ] [ Google Scholar ]
- 52. Hwang NS, Varghese S, Elisseeff J. Controlled differentiation of stem cells. Adv Drug Deliv Rev. 2007;60(2):199–214. doi: 10.1016/j.addr.2007.08.036. [ DOI ] [ PMC free article ] [ PubMed ] [ Google Scholar ]
- 53. Turner N, Grose R. Fibroblast growth factor signalling: from development to cancer. Nat Rev Cancer. 2010;10:116–129. doi: 10.1038/nrc2780. [ DOI ] [ PubMed ] [ Google Scholar ]
- 54. Rao TP, Kuhl M. An updated overview on Wnt signaling pathways: a prelude for more. Circ Res. 2010;106:1798–1806. doi: 10.1161/CIRCRESAHA.110.219840. [ DOI ] [ PubMed ] [ Google Scholar ]
- 55. Moustakas A, Heldin CH. The regulation of TGFbeta signal transduction. Development. 2009;136:3699–3714. doi: 10.1242/dev.030338. [ DOI ] [ PubMed ] [ Google Scholar ]
- 56. Efthymiou AG, Chen G, Rao M, Chen G, Boehm M. Self-renewal and cell lineage differentiation strategies in human embryonic stem cells and induced pluripotent stem cells. Expert Opin Biol Ther. 2014;14:1333–1344. doi: 10.1517/14712598.2014.922533. [ DOI ] [ PMC free article ] [ PubMed ] [ Google Scholar ]
- 57. Yang L, Soonpaa MH, Adler ED, Roepke TK, Kattman SJ, Kennedy M, Henckaerts E, Bonham K, Abbott GW, Linden RM, Field LJ, Keller GM. Human cardiovascular progenitor cells develop from a KDRþembryonic-stem-cell-derived population. Nature. 2008;453:524–528. doi: 10.1038/nature06894. [ DOI ] [ PubMed ] [ Google Scholar ]
- 58. Kroon E, Martinson LA, Kadoya K, Bang AG, Kelly OG, Eliazer S, Young H, Richardson M, Smart NG, Cunningham J, Agulnick AD, D’amour KA, Carpenter MK, Baetge EE. Pancreatic endoderm derived from human embryonic stem cells generates glucose-responsive insulin-secreting cells in vivo. Nat Biotechnol. 2008;26(4):443–452. doi: 10.1038/nbt1393. [ DOI ] [ PubMed ] [ Google Scholar ]
- 59. Vallier L, Reynolds D, Pedersen RA. Nodal inhibits differentiation of human embryonic stem cells along the neuroectodermal default pathway. Dev Biol. 2004;275:403–421. doi: 10.1016/j.ydbio.2004.08.031. [ DOI ] [ PubMed ] [ Google Scholar ]
- 60. Burridge PW, Zambidis ET. Highly efficient directed differentiation of human induced pluripotent stem cells into cardiomyocytes. Methods Mol Biol. 2013;997:149–161. doi: 10.1007/978-1-62703-348-0_12. [ DOI ] [ PubMed ] [ Google Scholar ]
- 61. Cai J, Zhao Y, Liu Y, Ye F, Song Z, Qin H, Meng S, Chen Y, Zhou R, Song X, Guo Y, Ding M, Deng H. Directed differentiation of human embryonic stem cells into functional hepatic cells. Hepatology. 2007;45:1229–1239. doi: 10.1002/hep.21582. [ DOI ] [ PubMed ] [ Google Scholar ]
- 62. Takasato M, Er PX, Becroft M, Vanslambrouck JM, Stanley EG, Elefanty AG, Little MH. Directing human embryonic stem cell differentiation towards a renal lineage generates a selforganizing kidney. Nat Cell Biol. 2014;16:118–126. doi: 10.1038/ncb2894. [ DOI ] [ PubMed ] [ Google Scholar ]
- 63. Huang SX, Islam MN, O’Neill J, Hu Z, Yang YG, Chen YW, Mumau M, Green MD, VunjakNovakovic G, Bhattacharya J, Snoeck HW. Efficient generation of lung and airway epithelial cells from human pluripotent stem cells. Nat Biotechnol. 2014;32:84–91. doi: 10.1038/nbt.2754. [ DOI ] [ PMC free article ] [ PubMed ] [ Google Scholar ]
- 64. Kadzik RS, Morrisey EE. Directing lung endoderm differentiation in pluripotent stem cells. Cell Stem Cell. 2012;10:355–361. doi: 10.1016/j.stem.2012.03.013. [ DOI ] [ PMC free article ] [ PubMed ] [ Google Scholar ]
- 65. Wichterle H, Lieberam I, Porter JA, Jessell TM. Directed differentiation of embryonic stem cells into motor neurons. Cell. 2002;110:385–397. doi: 10.1016/s0092-8674(02)00835-8. [ DOI ] [ PubMed ] [ Google Scholar ]
- 66. Spence JR, Mayhew CN, Rankin SA, Kuhar MF, Vallance JE, Tolle K, Hoskins EE, Kalinichenko VV, Wells SI, Zorn AM, Shroyer NF, Wells JM. Directed differentiation of human pluripotent stem cells into intestinal tissue in vitro. Nature. 2011;470:105–109. doi: 10.1038/nature09691. [ DOI ] [ PMC free article ] [ PubMed ] [ Google Scholar ]
- 67. Oldershaw RA, Baxter MA, Lowe ET, Bates N, Grady LM, Soncin F, Brison DR, Hardingham TE, Kimber SJ. Directed differentiation of human embryonic stem cells toward chondrocytes. Nat Biotechnol. 2010;28:1187–1194. doi: 10.1038/nbt.1683. [ DOI ] [ PubMed ] [ Google Scholar ]
- 68. Jun Cai, Ann DeLaForest, Joseph Fisher, Amanda Urick, Thomas Wagner, Kirk Twaroski, Max Cayo, Masato Nagaoka, Stephen A Duncan. Protocol for directed differentiation of human pluripotent stem cells toward a hepatocyte fate. 2012. DOI: 10.3824/stembook.1.52.1. [ PubMed ]
- 69. Frank-Kamenetsky M, Zhang XM, Bottega S, Guicherit O, Wichterle H, Dudek H, Bumcrot D, Wang FY, Jones S, Shulok J, Rubin LL, Porter JA. Small-molecule modulators of hedgehog signaling: identification and characterization of smoothened agonists and antagonists. J Biol. 2002;1:10. doi: 10.1186/1475-4924-1-10. [ DOI ] [ PMC free article ] [ PubMed ] [ Google Scholar ]
- 70. Oshima K, Shin K, Diensthuber M, Peng AW, Ricci AJ, Heller S. Mechanosensitive hair celllike cells from embryonic and induced pluripotent stem cells. Cell. 2010;141:704–716. doi: 10.1016/j.cell.2010.03.035. [ DOI ] [ PMC free article ] [ PubMed ] [ Google Scholar ]
- 71. Osakada F, Jin ZB, Hirami Y, Ikeda H, Danjyo T, Watanabe K, Sasai Y, Takahashi M. In vitro differentiation of retinal cells from human pluripotent stem cells by small-molecule induction. J Cell Sci. 2009;122:3169–3179. doi: 10.1242/jcs.050393. [ DOI ] [ PubMed ] [ Google Scholar ]
- 72. Kshitiz PJ, Kim P, Helen W, Engler AJ, Levchenko A, Kim DH. Control of stem cell fate and function by engineering physical microenvironments. Intergr Biol (Camb). 2012;4:1008–1018. doi: 10.1039/c2ib20080e. [ DOI ] [ PMC free article ] [ PubMed ] [ Google Scholar ]
- 73. Amps K, Andrews PW, Anyfantis G, Armstrong L, Avery S, Baharvand H, Baker J, Baker D, Munoz MB, Beil S, Benvenisty N, Ben-Yosef D, Biancotti JC, Bosman A, Brena RM, Brison D, Caisander G, Camarasa MV, Chen J, ChiaoE CYM, Choo AB, Collins D, et al. Screening ethnically diverse human embryonic stem cells identifies a chromosome 20 minimal amplicon conferring growth advantage. Nat Biotechnol. 2011;29:1132–1144. doi: 10.1038/nbt.2051. [ DOI ] [ PMC free article ] [ PubMed ] [ Google Scholar ]
- 74. Nukaya D, Minami K, Hoshikawa R, Yokoi N, Seino S. Preferential gene expression and epigenetic memory of induced pluripotent stem cells derived from mouse pancreas. Genes Cells. 2015;20:367–381. doi: 10.1111/gtc.12227. [ DOI ] [ PubMed ] [ Google Scholar ]
- 75. Murdoch A, Braude P, Courtney A, Brison D, Hunt C, Lawford-Davies J, Moore H, Stacey G, Sethe S, Procurement Working Group Of National Clinical H, E. S. C. F, National Clinical H, E. S. C. F The procurement of cells for the derivation of human embryonic stem cell lines for therapeutic use: recommendations for good practice. Stem Cell Rev. 2012;8:91–99. doi: 10.1007/s12015-011-9288-9. [ DOI ] [ PubMed ] [ Google Scholar ]
- 76. Hewitson H, Wood V, Kadeva N, Cornwell G, Codognotto S, Stephenson E, Ilic D. Generation of KCL035 research grade human embryonic stem cell line carrying a mutation in HBB gene. Stem Cell Res. 2016;16:210–212. doi: 10.1016/j.scr.2016.01.007. [ DOI ] [ PMC free article ] [ PubMed ] [ Google Scholar ]
- 77. Daley GQ, Hyun I, Apperley JF, Barker RA, Benvenisty N, Bredenoord AL, Breuer CK, Caulfield T, Cedars MI, Frey-Vasconcells J, Heslop HE, Jin Y, Lee RT, Mccabe C, Munsie M, Murry CE, Piantadosi S, Rao M, Rooke HM, Sipp D, Studer L, Sugarman J, et al. Setting global standards for stem cell research and clinical translation: the 2016 ISSCR guidelines. Stem Cell Rep. 2016;6:787–797. doi: 10.1016/j.stemcr.2016.05.001. [ DOI ] [ PMC free article ] [ PubMed ] [ Google Scholar ]
- 78. Takahashi K, Yamanaka S. Induction of pluripotent stem cells from mouse embryonic and adult fibroblast cultures by defined factors. Cell. 2006;126(4):663–676. doi: 10.1016/j.cell.2006.07.024. [ DOI ] [ PubMed ] [ Google Scholar ]
- 79. Loh YH, Wu Q, Chew JL, Vega VB, Zhang W, Chen X, Bourque G, George J, Leong B, Liu J, et al. The Oct4 and Nanog transcription network regulates pluripotency in mouse embryonic stem cells. Nat Genet. 2006;38:431–440. doi: 10.1038/ng1760. [ DOI ] [ PubMed ] [ Google Scholar ]
- 80. Menasche P, Vanneaux V, Hagege A, Bel A, Cholley B, Cacciapuoti I, Parouchev A, Benhamouda N, Tachdjian G, Tosca L, Trouvin JH, Fabreguettes JR, Bellamy V, Guillemain R, SuberbielleBoissel C, Tartour E, Desnos M, Larghero J. Human embryonic stem cell-derived cardiac progenitors for severe heart failure treatment: first clinical case report. Eur Heart J. 2015;36:2011–2017. doi: 10.1093/eurheartj/ehv189. [ DOI ] [ PubMed ] [ Google Scholar ]
- 81. Schwartz SD, Regillo CD, Lam BL, Eliott D, Rosenfeld PJ, Gregori NZ, Hubschman JP, Davis JL, Heilwell G, Spirn M, Maguire J, Gay R, Bateman J, Ostrick RM, Morris D, Vincent M, Anglade E, Del Priore LV, Lanza R. Human embryonic stem cell-derived retinal pigment epithelium in patients with age-related macular degeneration and Stargardt’s macular dystrophy: follow-up of two open-label phase 1/2 studies. Lancet. 2015;385:509–516. doi: 10.1016/S0140-6736(14)61376-3. [ DOI ] [ PubMed ] [ Google Scholar ]
- 82. Ilic D, Ogilvie C. Concise review: human embryonic stem cells-what have we done? What are we doing? Where are we going? Stem Cells. 2017;35:17–25. doi: 10.1002/stem.2450. [ DOI ] [ PubMed ] [ Google Scholar ]
- 83. Rocha V, et al. Clinical use of umbilical cord blood hematopoietic stem cells. Biol Blood Marrow Transplant. 2006;12(1):34–4. [ DOI ] [ PubMed ]
- 84. Longo UG, Ronga M, Maffulli N. Sports Med Arthrosc 17:112–126. Achilles tendinopathy. Sports Med Arthrosc. 2009;17:112–126. doi: 10.1097/JSA.0b013e3181a3d625. [ DOI ] [ PubMed ] [ Google Scholar ]
- 85. Tempfer H, Lehner C, Grütz M, Gehwolf R, Traweger A. Biological augmentation for tendon repair: lessons to be learned from development, disease, and tendon stem cell research. In: Gimble J, Marolt D, Oreffo R, Redl H, Wolbank S, editors. Cell engineering and regeneration. Reference Series in Biomedical Engineering. Cham: Springer; 2017.
- 86. Goldring MB, Goldring SR. Osteoarthritis. J Cell Physiol. 2007;213:626–634. doi: 10.1002/jcp.21258. [ DOI ] [ PubMed ] [ Google Scholar ]
- 87. Widuchowski W, Widuchowski J, Trzaska T. Articular cartilage defects: study of 25,124 knee arthroscopies. Knee. 2007;14:177–182. doi: 10.1016/j.knee.2007.02.001. [ DOI ] [ PubMed ] [ Google Scholar ]
- 88. Li R, Lin Q-X, Liang X-Z, Liu G-B, et al. Stem cell therapy for treating osteonecrosis of the femoral head: from clinical applications to related basic research. Stem Cell Res Therapy. 2018;9:291. doi: 10.1186/s13287-018-1018-7. [ DOI ] [ PMC free article ] [ PubMed ] [ Google Scholar ]
- 89. Gangji V, De Maertelaer V, Hauzeur JP. Autologous bone marrow cell implantation in the treatment of non-traumatic osteonecrosis of the femoral head: five year follow-up of a prospective controlled study. Bone. 2011;49(5):1005–1009. doi: 10.1016/j.bone.2011.07.032. [ DOI ] [ PubMed ] [ Google Scholar ]
- 90. Zhao D, Cui D, Wang B, Tian F, Guo L, Yang L, et al. Treatment of early stage osteonecrosis of the femoral head with autologous implantation of bone marrow-derived and cultured mesenchymal stem cells. Bone. 2012;50(1):325–330. doi: 10.1016/j.bone.2011.11.002. [ DOI ] [ PubMed ] [ Google Scholar ]
- 91. Sen RK, Tripathy SK, Aggarwal S, Marwaha N, Sharma RR, Khandelwal N. Early results of core decompression and autologous bone marrow mononuclear cells instillation in femoral head osteonecrosis: a randomized control study. J Arthroplast. 2012;27(5):679–686. doi: 10.1016/j.arth.2011.08.008. [ DOI ] [ PubMed ] [ Google Scholar ]
- 92. Lapasset L, Milhavet O, Prieur A, Besnard E, Babled A, Aït-Hamou N, Leschik J, Pellestor F, Ramirez JM, De Vos J, Lehmann S, Lemaitre JM. Rejuvenating senescent and centenarian human cells by reprogramming through the pluripotent state. Genes Dev. 2011;25:2248–2253. doi: 10.1101/gad.173922.111. [ DOI ] [ PMC free article ] [ PubMed ] [ Google Scholar ]
- 93. Sahin E, Depinho RA. Linking functional decline of telomeres, mitochondria and stem cells during ageing. Nature. 2010;464:520–8. [ DOI ] [ PMC free article ] [ PubMed ]
- 94. Petkovich DA, Podolskiy DI, Lobanov AV, Lee SG, Miller RA, Gladyshev VN. Using DNA methylation profiling to evaluate biological age and longevity interventions. Cell Metab. 2017;25:954–960. doi: 10.1016/j.cmet.2017.03.016. [ DOI ] [ PMC free article ] [ PubMed ] [ Google Scholar ]
- 95. Gerontology, Rejuvenation by cell reprogramming: a new horizon in. Rodolfo G. Goya, Marianne Lehmann, Priscila Chiavellini, Martina Canatelli-Mallat, Claudia B. Hereñú and Oscar A. Brown. Stem Cell Res Therapy . 2018, 9:349. 10.1186/s13287-018-1075-y. [ DOI ] [ PMC free article ] [ PubMed ]
- 96. Ocampo A, Reddy P, Martinez-Redondo P, Platero-Luengo A, Hatanaka F, Hishida T, Li M, Lam D, Kurita M, Beyret E, Araoka T, Vazquez-Ferrer E, Donoso D, Roman JLXJ, Rodriguez-Esteban C, Nuñez G, Nuñez Delicado E, Campistol JM, Guillen I, Guillen P, Izpisua In vivo amelioration of age-associated hallmarks by partial reprogramming. Cell. 2016;167:1719–1733. doi: 10.1016/j.cell.2016.11.052. [ DOI ] [ PMC free article ] [ PubMed ] [ Google Scholar ]
- 97. de Lázaro I, Cossu G, Kostarelos K. Transient transcription factor (OSKM) expression is key towards clinical translation of in vivo cell reprogramming. EMBO Mol Med. 2017;9:733–736. doi: 10.15252/emmm.201707650. [ DOI ] [ PMC free article ] [ PubMed ] [ Google Scholar ]
- 98. Sun S, Li ZQ, Glencer P, Cai BC, Zhang XM, Yang J, Li XR. Bringing the age-related macular degeneration high-risk allele age-related maculopathy susceptibility 2 into focus with stem cell technology. Stem Cell Res Ther. 2017;8:135. doi: 10.1186/s13287-017-0584-4. [ DOI ] [ PMC free article ] [ PubMed ] [ Google Scholar ]
- 99. Liu J. Induced pluripotent stem cell-derived neural stem cells: new hope for stroke? Stem Cell Res Ther. 2013;4:115. doi: 10.1186/scrt326. [ DOI ] [ PMC free article ] [ PubMed ] [ Google Scholar ]
- 100. Shahjalal HM, Dayem AA, Lim KM, Jeon TI, Cho SG. Generation of pancreatic β cells for treatment of diabetes: advances and challenges. Stem Cell ResTher. 2018;9:355. doi: 10.1186/s13287-018-1099-3. [ DOI ] [ PMC free article ] [ PubMed ] [ Google Scholar ]
- 101. Kroon E, Martinson LA, et al. Pancreatic endoderm derived from human embryonic stem cells generates glucose-responsive insulin-secreting cells in vivo. Nat Biotechnol. 2008;26;443–52. [ DOI ] [ PubMed ]
- 102. Arora V, Pooja A, Munshi AK. Banking stem cells from human exfoliated deciduous teeth. J Clin Pediatr Dent. 2009;33(4):289–294. doi: 10.17796/jcpd.33.4.y887672r0j703654. [ DOI ] [ PubMed ] [ Google Scholar ]
- 103. Mao JJ. Stem cells and the future of dental care. New York State Dental J. 2008;74(2):21–24. [ PubMed ] [ Google Scholar ]
- 104. Reznick, Jay B. Continuing education: stem cells: emerging medical and dental therapies for the dental Professional. Dentaltown Magazine . 2008, pp. 42–53.
- 105. Arthur A, Rychkov G, Shi S, Koblar SA, Gronthos S. Adult human dental pulp stem cells differentiate toward functionally active neurons under appropriate environmental cues. Stem Cells. 2008;26(7):1787–1795. doi: 10.1634/stemcells.2007-0979. [ DOI ] [ PubMed ] [ Google Scholar ]
- 106. Cordeiro MM, Dong Z, Kaneko T, Zhang Z, Miyazawa M, Shi S, Smith A. Dental pulp tissue engineering with stem cells from exfoliated. J Endod. 2008;34(8):962–969. doi: 10.1016/j.joen.2008.04.009. [ DOI ] [ PubMed ] [ Google Scholar ]
- 107. Hayashi K, Ohta H, Kurimoto K, Aramaki S, Saitou M. Reconstitution of the mouse germ cell specification pathway in culture by pluripotent stem cells. Cell. 2011;146(4):519–532. doi: 10.1016/j.cell.2011.06.052. [ DOI ] [ PubMed ] [ Google Scholar ]
- 108. Sadri-Ardekani H, Atala A. Testicular tissue cryopreservation and spermatogonial stem cell transplantation to restore fertility: from bench to bedside. Stem Cell ResTher. 2014;5:68. doi: 10.1186/scrt457. [ DOI ] [ PMC free article ] [ PubMed ] [ Google Scholar ]
- 109. Zhang Q, Xu M, Yao X, Li T, Wang Q, Lai D. Human amniotic epithelial cells inhibit granulosa cell apoptosis induced by chemotherapy and restore the fertility. Stem Cell Res Ther. 2015;6:152. doi: 10.1186/s13287-015-0148-4. [ DOI ] [ PMC free article ] [ PubMed ] [ Google Scholar ]
- 110. Ma DK, Bonaguidi MA, Ming GL, Song H. Adult neural stem cells in the mammalian central nervous system. Cell Res. 2009;19:672–682. doi: 10.1038/cr.2009.56. [ DOI ] [ PMC free article ] [ PubMed ] [ Google Scholar ]
- 111. Dantuma E, Merchant S, Sugaya K. Stem cells for the treatment of neurodegenerative diseases. Stem Cell ResTher. 2010;1:37. doi: 10.1186/scrt37. [ DOI ] [ PMC free article ] [ PubMed ] [ Google Scholar ]
- 112. Wang Q, Matsumoto Y, Shindo T, Miyake K, Shindo A, Kawanishi M, Kawai N, Tamiya T, Nagao S. Neural stem cells transplantation in cortex in a mouse model of Alzheimer’s disease. J Med Invest. 2006;53:61–69. doi: 10.2152/jmi.53.61. [ DOI ] [ PubMed ] [ Google Scholar ]
- 113. Moghadam FH, Alaie H, Karbalaie K, Tanhaei S, Nasr Esfahani MH, Baharvand H. Transplantation of primed or unprimed mouse embryonic stem cell-derived neural precursor cells improves cognitive function in Alzheimerian rats. Differentiation. 2009;78:59–68. doi: 10.1016/j.diff.2009.06.005. [ DOI ] [ PubMed ] [ Google Scholar ]
- 114. Byrne JA. Developing neural stem cell-based treatments for neurodegenerative diseases. Stem Cell ResTher. 2014;5:72. doi: 10.1186/scrt461. [ DOI ] [ PMC free article ] [ PubMed ] [ Google Scholar ]
- 115. Awe JP, Lee PC, Ramathal C, Vega-Crespo A, Durruthy-Durruthy J, Cooper A, Karumbayaram S, Lowry WE, Clark AT, Zack JA, Sebastiano V, Kohn DB, Pyle AD, Martin MG, Lipshutz GS, Phelps PE, Pera RA, Byrne JA. Generation and characterization of transgene-free human induced pluripotent stem cells and conversion to putative clinical-grade status. Stem Cell Res Ther. 2013;4:87. doi: 10.1186/scrt246. [ DOI ] [ PMC free article ] [ PubMed ] [ Google Scholar ]
- 116. Peng J, Zeng X. The role of induced pluripotent stem cells in regenerative medicine: neurodegenerative diseases. Stem Cell ResTher. 2011;2:32. doi: 10.1186/scrt73. [ DOI ] [ PMC free article ] [ PubMed ] [ Google Scholar ]
- 117. Wright BL, Barker RA. Established and emerging therapies for Huntington’s disease. 2007;7(6):579–87 https://www.ncbi.nlm.nih.gov/pubmed/17896994/579-87 . [ DOI ] [ PubMed ]
- 118. Lin NH, Gronthos S, Bartold PM. Stem cells and periodontal regeneration. Aust Dent J. 2008;53:108–121. doi: 10.1111/j.1834-7819.2008.00019.x. [ DOI ] [ PubMed ] [ Google Scholar ]
- 119. Seo BM, Miura M, Gronthos S, Bartold PM, Batouli S, Brahim J, et al. Investigation of multipotent postnatal stem cells from human periodontal ligament. Lancet. 2004;364:149–155. doi: 10.1016/S0140-6736(04)16627-0. [ DOI ] [ PubMed ] [ Google Scholar ]
- 120. Ramseier CA, Abramson ZR, Jin Q, Giannobile WV. Gene therapeutics for periodontal regenerative medicine. Dent Clin North Am. 2006;50:245–263. doi: 10.1016/j.cden.2005.12.001. [ DOI ] [ PMC free article ] [ PubMed ] [ Google Scholar ]
- 121. Shi S, Bartold PM, Miura M, Seo BM, Robey PG, Gronthos S. The efficacy of mesenchymal stem cells to regenerate and repair dental structures. OrthodCraniofac Res. 2005;8:191–199. doi: 10.1111/j.1601-6343.2005.00331.x. [ DOI ] [ PubMed ] [ Google Scholar ]
- 122. Iohara K, Nakashima M, Ito M, Ishikawa M, Nakasima A, Akamine A. Dentin regeneration by dental pulp stem cell therapy with recombinant human bone morphogenetic protein. J Dent Res. 2004;83:590–595. doi: 10.1177/154405910408300802. [ DOI ] [ PubMed ] [ Google Scholar ]
- 123. Hu B, Unda F, Bopp-Kuchler S, Jimenez L, Wang XJ, Haikel Y, et al. Bone marrow cells can give rise to ameloblast-like cells. J Dent Res. 2006;85:416–421. doi: 10.1177/154405910608500504. [ DOI ] [ PubMed ] [ Google Scholar ]
- 124. Liu Y, Liu W, Hu C, Xue Z, Wang G, Ding B, Luo H, Tang L, Kong X, Chen X, Liu N, Ding Y, Jin Y. MiR-17 modulates osteogenic differentiation through a coherent feed-forward loop in mesenchymal stem cells isolated from periodontal ligaments of patients with periodontitis. Stem Cells. 2011;29(11):1804–1816. doi: 10.1002/stem.728. [ DOI ] [ PubMed ] [ Google Scholar ]
- 125. Raspini G, Wolff J, Helminen M, Raspini G, Raspini M, Sándor GK. Dental stem cells harvested from third molars combined with bioactive glass can induce signs of bone formation in vitro. J Oral Maxillofac Res. 2018;9(1):e2. Published 2018 Mar 31. 10.5037/jomr.2018.9102. [ DOI ] [ PMC free article ] [ PubMed ]
- 126. Christodoulou I, Goulielmaki M, Devetzi M, Panagiotidis M, Koliakos G, Zoumpourlis V. Mesenchymal stem cells in preclinical cancer cytotherapy: a systematic review. Stem Cell Res Ther. 2018;9(1;336). 10.1186/s13287-018-1078-8. [ DOI ] [ PMC free article ] [ PubMed ]
- 127. Bansal R, Jain A. Current overview on dental stem cells applications in regenerative dentistry. J Nat Sci Biol Med. 2015;6(1):29–34. doi: 10.4103/0976-9668.149074. [ DOI ] [ PMC free article ] [ PubMed ] [ Google Scholar ]
- 128. Edgar Ledesma-Martínez, Víctor Manuel Mendoza-Núñez, Edelmiro Santiago-Osorio. Mesenchymal stem cells derived from dental pulp: a review. Stem Cells Int . 2016, 4,709,572, p. doi: 10.1155/2016/4709572]. [ DOI ] [ PMC free article ] [ PubMed ]
- 129. Grzech-Leśniak K. Making use of lasers in periodontal treatment: a new gold standard? Photomed Laser Surg. 2017;35(10):513–514. doi: 10.1089/pho.2017.4323. [ DOI ] [ PubMed ] [ Google Scholar ]
- 130. Miura M, Gronthos S, Zhao M, Lu B, Fisher LW, Robey PG, Shi S. SHED: stem cells from human exfoliated deciduous teeth. Proc Natl Acad Sci U S A. 2003;100(10):5807–5812. doi: 10.1073/pnas.0937635100. [ DOI ] [ PMC free article ] [ PubMed ] [ Google Scholar ]
- 131. Yasui T, Mabuchi Y, Toriumi H, Ebine T, Niibe K, Houlihan DD, Morikawa S, Onizawa K, Kawana H, Akazawa C, Suzuki N, Nakagawa T, Okano H, Matsuzaki Y. Purified human dental pulp stem cells promote osteogenic regeneration. J Dent Res. 2016;95(2):206–214. doi: 10.1177/0022034515610748. [ DOI ] [ PubMed ] [ Google Scholar ]
- 132. Yamamoto A, Sakai K, Matsubara K, Kano F, Ueda M. Multifaceted neuro-regenerative activities of human dental pulp stem cells for functional recovery after spinal cord injury. Neurosci Res. 2014;78:16–20. doi: 10.1016/j.neures.2013.10.010. [ DOI ] [ PubMed ] [ Google Scholar ]
- 133. d’Aquino R, De Rosa A, Lanza V, Tirino V, Laino L, Graziano A, Desiderio V, Laino G, Papaccio G. Human mandible bone defect repair by the grafting of dental pulp stem/progenitor cells and collagen sponge biocomplexes. Eur Cell Mater. 2009;12:75–83. doi: 10.22203/ecm.v018a07. [ DOI ] [ PubMed ] [ Google Scholar ]
- 134. Wang L, Shen H, Zheng W, Tang L, Yang Z, Gao Y, Yang Q, Wang C, Duan Y, Jin Y. Characterization of stem cells from alveolar periodontal ligament. Tissue Eng. Part A. 2011;17(7–8):1015–1026. doi: 10.1089/ten.tea.2010.0140. [ DOI ] [ PubMed ] [ Google Scholar ]
- 135. Iwata T, Yamato M, Zhang Z, Mukobata S, Washio K, Ando T, Feijen J, Okano T, Ishikawa I. Validation of human periodontal ligament-derived cells as a reliable source for cytotherapeutic use. J Clin Periodontol. 2010;37(12):1088–1099. doi: 10.1111/j.1600-051X.2010.01597.x. [ DOI ] [ PubMed ] [ Google Scholar ]
- 136. Chen F-M, Gao L-N, Tian B-M, Zhang X-Y, Zhang Y-J, Dong G-Y, Lu H, et al. Treatment of periodontal intrabony defects using autologous periodontal ligament stem cells: a randomized clinical trial. Stem Cell Res Ther. 2016;7:33. doi: 10.1186/s13287-016-0288-1. [ DOI ] [ PMC free article ] [ PubMed ] [ Google Scholar ]
- 137. Bakopoulou A, Leyhausen G, Volk J, Tsiftsoglou A, Garefis P, Koidis P, Geurtsen W. Comparative analysis of in vitro osteo/odontogenic differentiation potential of human dental pulp stem cells (DPSCs) and stem cells from the apical papilla (SCAP) Arch Oral Biol. 2011;56(7):709–721. doi: 10.1016/j.archoralbio.2010.12.008. [ DOI ] [ PubMed ] [ Google Scholar ]
- 138. Han C, Yang Z, Zhou W, Jin F, Song Y, Wang Y, Huo N, Chen L, Qian H, Hou R, Duan Y, Jin Y. Periapical follicle stem cell: a promising candidate for cementum/periodontal ligament regeneration and bio-root engineering. Stem Cells Dev. 2010;19(9):1405–1415. doi: 10.1089/scd.2009.0277. [ DOI ] [ PubMed ] [ Google Scholar ]
- 139. Luan X, Ito Y, Dangaria S, Diekwisch TG. Dental follicle progenitor cell heterogeneity in the developing mouse periodontium. Stem Cells Dev. 2006;15(4):595–608. doi: 10.1089/scd.2006.15.595. [ DOI ] [ PMC free article ] [ PubMed ] [ Google Scholar ]
- 140. Handa K, Saito M, Tsunoda A, Yamauchi M, Hattori S, Sato S, Toyoda M, Teranaka T, Narayanan AS. Progenitor cells from dental follicle are able to form cementum matrix in vivo. Connect Tissue Res. 2002;43(2–3):406–408. doi: 10.1080/03008200290001023. [ DOI ] [ PubMed ] [ Google Scholar ]
- 141. Guo W, Chen L, Gong K, Ding B, Duan Y, Jin Y. Heterogeneous dental follicle cells and the regeneration of complex periodontal tissues. Tissue Engineering. Part A. 2012;18(5–6):459–470. doi: 10.1089/ten.tea.2011.0261. [ DOI ] [ PMC free article ] [ PubMed ] [ Google Scholar ]
- 142. Bai, Yudi et al. Cementum- and periodontal ligament-like tissue formation by dental follicle cell sheets co-cultured with Hertwig’s epithelial root sheath cells. Bone. 2011, 48, Issue 6, pp. 1417–1426, 10.1016/j.bone.2011.02.016. [ DOI ] [ PubMed ]
- 143. Cordeiro MM, Dong Z, Kaneko T, Zhang Z, Miyazawa M, Shi S, et al. Dental pulp tissue engineering with stem cells from exfoliated deciduous teeth. 2008, 34, pp. 962–969. [ DOI ] [ PubMed ]
- 144. Dobie K, Smith G, Sloan AJ, Smith AJ. Effects of alginate, hydrogels and TGF-beta 1 on human dental pulp repair in vitro. Connect Tissue Res 2. 2002;43:387–390. doi: 10.1080/03008200290000574. [ DOI ] [ PubMed ] [ Google Scholar ]
- 145. Friedlander LT, Cullinan MP, Love RM. Dental stem cells and their potential role in apexogenesis and apexification. Int Endod J. 2009;42:955–962. doi: 10.1111/j.1365-2591.2009.01622.x. [ DOI ] [ PubMed ] [ Google Scholar ]
- 146. Cai J, Zhang Y, Liu P, Chen S, Wu X, Sun Y, Li A, Huang K, Luo R, Wang L, Liu Y, Zhou T, Wei S, Pan G, Pei D, Generation of tooth-like structures from integration-free human urine induced pluripotent stem cells. Cell Regen (Lond). July 30, 2013, 2(1), pp. 6, doi: 10.1186/2045-9769-2-6. [ DOI ] [ PMC free article ] [ PubMed ]
- 147. Craig J. Taylor, Eleanor M. Bolton, and J. Andrew Bradley 2011 Aug 12 and 10.1098/rstb.2011.0030], 366(1575): 2312–2322. [doi:. Immunological considerations for embryonic and induced pluripotent stem cell banking,. Philos Trans R SocLond B Biol Sci. 2011, 366(1575), pp. 2312–2322, doi:10.1098/rstb.2011.0030. [ DOI ] [ PMC free article ] [ PubMed ]
- 148. T.R. Nayak, H. Andersen, V.S. Makam, C. Khaw, S. Bae, X.F. Xu, P.L.R. Ee, J.H. Ahn, B.H. Hong, G. Pastorin, B. Ozyilmaz, ACS Nano, 5 (6) (2011), pp. 4. Graphene for controlled and accelerated osteogenic differentiation of human mesenchymal stem cells,. ACS Nano. 2011, pp. 4670–4678. [ DOI ] [ PubMed ]
- 149. Lee WC, Lim C, Shi H, Tang LAL, Wang Y, Lim CT, Loh KP. Origin of enhanced stem cell growth and differentiation on graphene and graphene oxide. ACS Nano. 2011;5(9):7334–7341. doi: 10.1021/nn202190c. [ DOI ] [ PubMed ] [ Google Scholar ]
- 150. Kenry LWC, Loh KP, Lim CT. When stem cells meet graphene: opportunities and challenges in regenerative medicine. Biomaterials. 2018;155:236–250. doi: 10.1016/j.biomaterials.2017.10.004. [ DOI ] [ PubMed ] [ Google Scholar ]
- 151. Yuan A, Farber EL, Rapoport AL, Tejada D, Deniskin R, Akhmedov NB, et al. Transfer of microRNAs by embryonic stem cell microvesicles. 2009. 2009, 4(3), p. 10.1371/journal.pone. 0004722. [ DOI ] [ PMC free article ] [ PubMed ]
- 152. Oh, Myeongsik, et al. Exosomes derived from human induced pluripotent stem cells ameliorate the aging of skin fibroblasts. Int. J. Mol. Sci. 2018, 19(6), p. 1715. [ DOI ] [ PMC free article ] [ PubMed ]
- 153. Ramirez MI. et al. Technical challenges of working with extracellular vesicles. Nanoscale. 2018;10:881–906. doi: 10.1039/c7nr08360b. [ DOI ] [ PubMed ] [ Google Scholar ]
- 154. Valadi H, et al. Exosome-mediated transfer of mRNAs and microRNAs is a novel mechanism of genetic exchange between cells. Nat. Cell Biol. 2007;9:654–659. doi: 10.1038/ncb1596. [ DOI ] [ PubMed ] [ Google Scholar ]
- 155. Mateescu B, et al. Obstacles and opportunities in the functional analysis of extracellular vesicle RNA—an ISEV position paper. J. Extracell. Vesicles. 2017;6(1). 10.1080/20013078.2017.1286095. [ DOI ] [ PMC free article ] [ PubMed ]
- 156. Nawaz M, et al. Extracellular vesicles: evolving factors in stem cell biology. Stem Cells Int. 2016;2016:17. Article ID 1073140. [ DOI ] [ PMC free article ] [ PubMed ]
- 157. Helfrich, Y.R., Sachs, D.L. and Voorhees, J.J. Overview of skin aging and photoaging. Dermatol. Nurs. 20, pp. 177–183, https://www.ncbi.nlm.nih.gov/pubmed/18649702 . [ PubMed ]
- 158. Julia Tigges, Jean Krutmann, Ellen Fritsche, Judith Haendeler, Heiner Schaal, Jens W. Fischer, Faiza Kalfalah, Hans Reinke, Guido Reifenberger, Kai Stühler, Natascia Ventura, Sabrina Gundermann, Petra Boukamp, Fritz Boege. The hallmarks of fibroblast ageing, mechanisms of ageing and development, 138, 2014, Pages 26–44. 2014, 138, pp. 26–44, ISSN 0047–6374, 10.1016/j.mad.2014.03.004. [ DOI ] [ PubMed ]
- 159. Huh MI, Kim MS, Kim HK, et al. Effect of conditioned media collected from human amniotic fluid-derived stem cells (hAFSCs) on skin regeneration and photo-aging. Tissue Eng Regen Med. 2014;11:171. [ Google Scholar ]
- 160. Togel F, Hu Z, Weiss K, et al. Administered mesenchymal stem cells protect against ischemic acute renal failure through differentiation-independent mechanisms. Am J Physiol Renal Physiol. 2005;289:F31. doi: 10.1152/ajprenal.00007.2005. [ DOI ] [ PubMed ] [ Google Scholar ]
- 161. Liu J, Han G, Liu H, et al. Suppression of cholangiocarcinoma cell growth by human umbilical cord mesenchymal stem cells: a possible role of Wnt and Akt signaling. PLoS One. 2013;8:e62844. doi: 10.1371/journal.pone.0062844. [ DOI ] [ PMC free article ] [ PubMed ] [ Google Scholar ]
- 162. Oh M, et al. Promotive effects of human induced pluripotent stem cell-conditioned medium on the proliferation and migration of dermal fibroblasts. Biotechnol. Bioprocess Eng. 2017;22:561–568. [ Google Scholar ]
- 163. Chen L, Tredget EE, Wu PY, Wu Y. Paracrine factors of mesenchymal stem cells recruit macrophages and endothelial lineage cells and enhance wound healing. PloS One. 2008;3:e1886. doi: 10.1371/journal.pone.0001886. [ DOI ] [ PMC free article ] [ PubMed ] [ Google Scholar ]
- 164. Bae J-S, Lee S-H, Kim J-E, Choi J-Y, Park R-W, Park JY, Park H-S, Sohn Y-S, Lee D-S, Lee EB. βig-h3 supports keratinocyte adhesion, migration, and proliferation through α3β1 integrin. Biochem. Biophys. Res. Commun. 2002;294:940–948. doi: 10.1016/S0006-291X(02)00576-4. [ DOI ] [ PubMed ] [ Google Scholar ]
- 165. Zhou B-R, Xu Y, Guo S-L, Xu Y, Wang Y, Zhu F, Permatasari F, Wu D, Yin Z-Q, Luo D. The effect of conditioned media of adipose-derived stem cells on wound healing after ablative fractional carbon dioxide laser resurfacing. BioMed Res. Int. 2013;519:126. doi: 10.1155/2013/519126. [ DOI ] [ PMC free article ] [ PubMed ] [ Google Scholar ]
- 166. Peng Y, Baulier E, Ke Y, Young A, Ahmedli NB, Schwartz SD, et al. Human embryonic stem cells extracellular vesicles and their effects on immortalized human retinal Müller cells. PLoS ONE. 2018, 13(3), p. 10.1371/journal.pone.019400. [ DOI ] [ PMC free article ] [ PubMed ]
- 167. Harris MT, Butler DL, Boivin GP, Florer JB, Schantz EJ, Wenstrup RJ. Mesenchymal stem cells used for rabbit tendon repair can form ectopic bone and express alkaline phosphatase activity in constructs. J Orthop Res. 2004;22:998–1003. doi: 10.1016/j.orthres.2004.02.012. [ DOI ] [ PubMed ] [ Google Scholar ]
- 168. Mascetti VL, Pedersen RA. Human-mouse chimerism validates human stem cell pluripotency. Cell Stem Cell. 2016;18:67–72. doi: 10.1016/j.stem.2015.11.017. [ DOI ] [ PMC free article ] [ PubMed ] [ Google Scholar ]
- 169. Gandia C, Armiñan A, García-Verdugo JM, Lledó E, Ruiz A, Miñana MD, Sanchez-Torrijos J, Payá R, Mirabet V, Carbonell-Uberos F, Llop M, Montero JA, Sepúlveda P. Human dental pulp stem cells improve left ventricular function, induce angiogenesis, and reduce infarct size in rats with acute myocardial infarction. Stem Cells. 2007;26(3):638–645. doi: 10.1634/stemcells.2007-0484. [ DOI ] [ PubMed ] [ Google Scholar ]
- 170. Perry BC, Zhou D, Wu X, Yang FC, Byers MA, Chu TM, Hockema JJ, Woods EJ, Goebel WS. Collection, cryopreservation, and characterization of human dental pulp-derived mesenchymal stem cells for banking and clinical use. Tissue Eng Part C Methods. 2008;14(2):149–156. doi: 10.1089/ten.tec.2008.0031. [ DOI ] [ PMC free article ] [ PubMed ] [ Google Scholar ]
- 171. Garcia-Olmo D, Garcia-Arranz M, Herreros D, et al. A phase I clinical trial of the treatment of Crohn’s fistula by adipose mesenchymal stem cell transplantation. Dis Colon Rectum. 2005;48:1416–1423. doi: 10.1007/s10350-005-0052-6. [ DOI ] [ PubMed ] [ Google Scholar ]
- 172. de Mendonça CA, Bueno DF, Martins MT, Kerkis I, Kerkis A, Fanganiello RD, Cerruti H, Alonso N, Passos-Bueno MR. Reconstruction of large cranial defects in nonimmunosuppressed experimental design with human dental pulp stem cells. J Craniofac Surg. 2008;19(1):204–210. doi: 10.1097/scs.0b013e31815c8a54. [ DOI ] [ PubMed ] [ Google Scholar ]
- 173. Seo BM, Sonoyama W, Yamaza T, Coppe C, Kikuiri T, Akiyama K, Lee JS, Shi S. SHED repair critical-size calvarial defects in mice. Oral Dis. 2008;14(5):428–434. doi: 10.1111/j.1601-0825.2007.01396.x. [ DOI ] [ PMC free article ] [ PubMed ] [ Google Scholar ]
- 174. Abbas, Diakonov I., Sharpe P. Neural crest origin of dental stem cells. Pan European Federation of the International Association for Dental Research (PEF IADR). 2008, Vols. Seq #96 - Oral Stem Cells.
- 175. Kerkis I, Ambrosio CE, Kerkis A, Martins DS, Gaiad TP, Morini AC, Vieira NM, Marina P, et al. Early transplantation of human immature dental pulp stem cells from baby teeth to golden retriever muscular dystrophy (GRMD) dogs. J Transl Med. 2008;6:35. doi: 10.1186/1479-5876-6-35. [ DOI ] [ PMC free article ] [ PubMed ] [ Google Scholar ]
- 176. Xianrui Yang, Li Li, Li Xiao, Donghui Zhang. Recycle the dental fairy’s package: overview of dental pulp stem cells. Stem Cell Res Ther . 2018, 9, 1, 1. 10.1186/s13287-018-1094-8. [ DOI ] [ PMC free article ] [ PubMed ]
- 177. Wang J, Wang X, Sun Z, Wang X, Yang H, Shi S, Wang S. Stem cells from human-exfoliated deciduous teeth can differentiate into dopaminergic neuron-like cells. Stem Cells Dev. 2010;19:1375–1383. doi: 10.1089/scd.2009.0258. [ DOI ] [ PMC free article ] [ PubMed ] [ Google Scholar ]
- 178. Wang J, et al. The odontogenic differentiation of human dental pulp stem cells on nanofibrous poly (L-lactic acid) scaffolds in vitro and in vivo. Acta Biomater. 2010;6(10):3856–3863. doi: 10.1016/j.actbio.2010.04.009. [ DOI ] [ PMC free article ] [ PubMed ] [ Google Scholar ]
- 179. Davies OG, Cooper PR, Shelton RM, Smith AJ, Scheven BA. A comparison of the in vitro mineralisation and dentinogenic potential of mesenchymal stem cells derived from adipose tissue, bone marrow and dental pulp. J Bone Miner Metab. 2015;33:371–382. doi: 10.1007/s00774-014-0601-y. [ DOI ] [ PubMed ] [ Google Scholar ]
- 180. Huang GT-J, Shagramanova K, Chan SW. Formation of odontoblast-like cells from cultured human dental pulp cells on dentin in vitro. J Endod. 2006;32:1066–1073. doi: 10.1016/j.joen.2006.05.009. [ DOI ] [ PubMed ] [ Google Scholar ]
- 181. Shi S, Robey PG, Gronthos S. Comparison of human dental pulp and bone marrow stromal stem cells by cDNA microarray analysis. Bone. 2001;29(6):532–539. doi: 10.1016/s8756-3282(01)00612-3. [ DOI ] [ PubMed ] [ Google Scholar ]
- 182. Gronthos S, Mankani M, Brahim J, Robey PG, Shi S. Postnatal human dental pulp stem cells (DPSCs) in vitro and in vivo. Proc Natl Acad Sci U S A. 2000;97:13625–13630. doi: 10.1073/pnas.240309797. [ DOI ] [ PMC free article ] [ PubMed ] [ Google Scholar ]
- 183. Nuti N, Corallo C, Chan BMF, Ferrari M, Gerami-Naini B. Multipotent differentiation of human dental pulp stem cells: a literature review. Stem Cell Rev Rep. 2016;12:511–523. doi: 10.1007/s12015-016-9661-9. [ DOI ] [ PubMed ] [ Google Scholar ]
- 184. Ferro F, et al. Dental pulp stem cells differentiation reveals new insights in Oct4A dynamics. PloS One. 2012;7(7):e41774. [ DOI ] [ PMC free article ] [ PubMed ]
- 185. Conde MCM, Chisini LA, Grazioli G, Francia A, Carvalho RVd, Alcázar JCB, Tarquinio SBC, Demarco FF. Does cryopreservation affect the biological properties of stem cells from dental tissues? A systematic review. Braz Dent J. 2016;1210(6):633-40. 10.1590/0103-6440201600980. [ DOI ] [ PubMed ]
- 186. Papaccio G, Graziano A, d’Aquino R, Graziano MF, Pirozzi G, Menditti D, De Rosa A, Carinci F, Laino G. Long-term cryopreservation of dental pulp stem cells (SBP-DPSCs) and their differentiated osteoblasts: a cell source for tissue repair. J Cell Physiol. 2006;208:319–325. doi: 10.1002/jcp.20667. [ DOI ] [ PubMed ] [ Google Scholar ]
- 187. Alge DL, Zhou D, Adams LL, et. al. Donor-matched comparison of dental pulp stem cells and bone marrow-derived mesenchymal stem cells in a rat model. J Tissue Eng Regen Med. 2010;4(1):73–81. [ DOI ] [ PMC free article ] [ PubMed ]
- 188. Jo Y-Y, Lee H-J, Kook S-Y, Choung H-W, Park J-Y, Chung J-H, Choung Y-H, Kim E-S, Yang H-C, Choung P-H. Isolation and characterization of postnatal stem cells from human dental tissues. Tissue Eng. 2007;13:767–773. doi: 10.1089/ten.2006.0192. [ DOI ] [ PubMed ] [ Google Scholar ]
- 189. Gronthos S, Brahim J, Li W, Fisher LW, Cherman N, Boyde A, DenBesten P, Robey PG, Shi S. Stem cell properties of human dental pulp stem cells. J Dent Res. 2002;81:531–535. doi: 10.1177/154405910208100806. [ DOI ] [ PubMed ] [ Google Scholar ]
- 190. Laino G, d’Aquino R, Graziano A, Lanza V, Carinci F, Naro F, Pirozzi G, Papaccio G. A new population of human adult dental pulp stem cells: a useful source of living autologous fibrous bone tissue (LAB) J Bone Miner Res. 2005;20:1394–1402. doi: 10.1359/JBMR.050325. [ DOI ] [ PubMed ] [ Google Scholar ]
- 191. Zainal A, Shahrul H, et al. In vitro chondrogenesis transformation study of mouse dental pulp stem cells. Sci World J. 2012;2012:827149. [ DOI ] [ PMC free article ] [ PubMed ]
- 192. Wei X, et al. Expression of mineralization markers in dental pulp cells. J Endod. 2007;33(6):703–708. doi: 10.1016/j.joen.2007.02.009. [ DOI ] [ PubMed ] [ Google Scholar ]
- 193. Dai J, et al. The effect of co-culturing costal chondrocytes and dental pulp stem cells combined with exogenous FGF9 protein on chondrogenesis and ossification in engineered cartilage. Biomaterials. 2012;33(31):7699–7711. doi: 10.1016/j.biomaterials.2012.07.020. [ DOI ] [ PubMed ] [ Google Scholar ]
- 194. Vasandan AB, et al. Functional differences in mesenchymal stromal cells from human dental pulp and periodontal ligament. J Cell Mol Med. 2014;18(2):344–354. doi: 10.1111/jcmm.12192. [ DOI ] [ PMC free article ] [ PubMed ] [ Google Scholar ]
- 195. Werle SB, et al. Carious deciduous teeth are a potential source for dental pulp stem cells. Clin Oral Investig. 2015;20:75–81. doi: 10.1007/s00784-015-1477-5. [ DOI ] [ PubMed ] [ Google Scholar ]
- 196. Nemeth CL, et al. Enhanced chondrogenic differentiation of dental pulp stem cells using nanopatterned PEG-GelMA-HA hydrogels. Tissue Eng A. 2014;20(21–22):2817–2829. doi: 10.1089/ten.tea.2013.0614. [ DOI ] [ PMC free article ] [ PubMed ] [ Google Scholar ]
- 197. Paino F, Ricci G, De Rosa A, D’Aquino R, Laino L, Pirozzi G, et al. Ecto-mesenchymal stem cells from dental pulp are committed to differentiate into active melanocytes. Eur. Cell Mater. 2010;20:295–305. doi: 10.22203/ecm.v020a24. [ DOI ] [ PubMed ] [ Google Scholar ]
- 198. Ferro F, Spelat R, Baheney CS. Dental pulp stem cell (DPSC) isolation, characterization, and differentiation. In: Kioussi C, editor. Stem cells and tissue repair. Methods in molecular biology (methods and protocols): Humana Press. 2014;1210. [ DOI ] [ PubMed ]
- 199. Ishkitiev N, Yaegaki K, Imai T, Tanaka T, Nakahara T, Ishikawa H, Mitev V, Haapasalo M. High-purity hepatic lineage differentiated from dental pulp stem cells in serum-free medium. J Endod. 2012;38:475–480. doi: 10.1016/j.joen.2011.12.011. [ DOI ] [ PubMed ] [ Google Scholar ]
Associated Data
This section collects any data citations, data availability statements, or supplementary materials included in this article.
Data Availability Statement
- View on publisher site
- PDF (1.8 MB)
- Collections
Similar articles
Cited by other articles, links to ncbi databases.
- Download .nbib .nbib
- Format: AMA APA MLA NLM
Add to Collections
Thank you for visiting nature.com. You are using a browser version with limited support for CSS. To obtain the best experience, we recommend you use a more up to date browser (or turn off compatibility mode in Internet Explorer). In the meantime, to ensure continued support, we are displaying the site without styles and JavaScript.
- View all journals
- Explore content
- About the journal
- Publish with us
- Sign up for alerts
- News & Views
- Published: 11 November 2024
Autologous stem-cell derived islets — the ultimate frontier in diabetes mellitus?
- A. M. James Shapiro ORCID: orcid.org/0000-0002-6215-0990 1
Nature Reviews Endocrinology ( 2024 ) Cite this article
238 Accesses
71 Altmetric
Metrics details
- Endocrinology
- Stem-cell research
A patient with longstanding type 1 diabetes mellitus has achieved insulin independence for at least 1 year after transplantation of autologous stem cell islets. These cells were differentiated from inducible pluripotent stem cells from adipose tissue and were transplanted into the rectus sheath of the abdominal wall.
This is a preview of subscription content, access via your institution
Access options
Access Nature and 54 other Nature Portfolio journals
Get Nature+, our best-value online-access subscription
24,99 € / 30 days
cancel any time
Subscribe to this journal
Receive 12 print issues and online access
195,33 € per year
only 16,28 € per issue
Buy this article
- Purchase on SpringerLink
- Instant access to full article PDF
Prices may be subject to local taxes which are calculated during checkout
Shapiro, A. M. et al. N. Engl. J. Med. 343 , 230–238 (2000).
Article PubMed CAS Google Scholar
Hering, B. J. et al. Diabetes Care 39 , 1230–1240 (2016).
Article PubMed PubMed Central CAS Google Scholar
Marfil-Garza, B. A. et al. Lancet Diabetes Endocrinol. 10 , 519–532 (2022).
Kroon, E. et al. Nat. Biotechnol. 26 , 443–452 (2008).
Shapiro, A. M. J. et al. Cell Rep. Med. 2 , 100466 (2021).
Keymeulen, B. et al. Nat. Biotechnol. 42 , 1507–1514 (2024).
Reichman, T. R., Ricordi, C., Naji, A. & Markmann, J. F. Diabetes 72 , 826-P (2023).
Article Google Scholar
Wang, S. et al. Cell 187 , 6152–6164.e18 (2024).
Verhoeff, K. et al. Transplantation 106 , 2224–2231 (2022).
Wu, J. et al. Cell Discov. 10 , 45 (2024).
Download references
Author information
Authors and affiliations.
Department of Surgery, Faculty of Medicine and Dentistry, University of Alberta, Edmonton, Alberta, Canada
A. M. James Shapiro
You can also search for this author in PubMed Google Scholar
Corresponding author
Correspondence to A. M. James Shapiro .
Ethics declarations
Competing interests.
A.M.J.S. is a consultant for Vertex Inc., Betalin Ltd and Aspect Biosystems Inc., and has previously consulted for ViaCyte Inc.
Rights and permissions
Reprints and permissions
About this article
Cite this article.
Shapiro, A.M.J. Autologous stem-cell derived islets — the ultimate frontier in diabetes mellitus?. Nat Rev Endocrinol (2024). https://doi.org/10.1038/s41574-024-01064-x
Download citation
Published : 11 November 2024
DOI : https://doi.org/10.1038/s41574-024-01064-x

Share this article
Anyone you share the following link with will be able to read this content:
Sorry, a shareable link is not currently available for this article.
Provided by the Springer Nature SharedIt content-sharing initiative
Quick links
- Explore articles by subject
- Guide to authors
- Editorial policies
Sign up for the Nature Briefing newsletter — what matters in science, free to your inbox daily.

- Open access
- Published: 06 November 2024
Emerging insights into epigenetics and hematopoietic stem cell trafficking in age-related hematological malignancies
- Yang Xinyi ORCID: orcid.org/0009-0009-2320-0834 1 ,
- Reshetov Igor Vladimirovich 1 ,
- Narasimha M. Beeraka 3 , 4 , 5 , 9 ,
- Allaka Satyavathi 8 ,
- Dinisha Kamble 5 ,
- Vladimir N. Nikolenko 3 ,
- Allaka Naga Lakshmi 6 ,
- Basappa Basappa 7 ,
- Padmanabha Reddy Y 4 ,
- Ruitai Fan 2 &
- Junqi Liu 2
Stem Cell Research & Therapy volume 15 , Article number: 401 ( 2024 ) Cite this article
560 Accesses
6 Altmetric
Metrics details
Hematopoiesis within the bone marrow (BM) is a complex and tightly regulated process predominantly influenced by immune factors. Aging, diabetes, and obesity are significant contributors to BM niche damage, which can alter hematopoiesis and lead to the development of clonal hematopoiesis of intermediate potential (CHIP). Genetic/epigenetic alterations during aging could influence BM niche reorganization for hematopoiesis or clonal hematopoiesis. CHIP is driven by mutations in genes such as Tet2, Dnmt3a, Asxl1, and Jak2, which are associated with age-related hematological malignancies.
This literature review aims to provide an updated exploration of the functional aspects of BM niche cells within the hematopoietic microenvironment in the context of age-related hematological malignancies. The review specifically focuses on how immunological stressors modulate different signaling pathways that impact hematopoiesis. Methods: An extensive review of recent studies was conducted, examining the roles of various BM niche cells in hematopoietic stem cell (HSC) trafficking and the development of age-related hematological malignancies. Emphasis was placed on understanding the influence of immunological stressors on these processes.
Recent findings reveal a significant microheterogeneity and temporal stochasticity of niche cells across the BM during hematopoiesis. These studies demonstrate that niche cells, including mesenchymal stem cells, osteoblasts, and endothelial cells, exhibit dynamic interactions with HSCs, significantly influenced by the BM microenvironment as the age increases. Immunosurveillance plays a crucial role in maintaining hematopoietic homeostasis, with alterations in immune signaling pathways contributing to the onset of hematological malignancies. Novel insights into the interaction between niche cells and HSCs under stress/aging conditions highlight the importance of niche plasticity and adaptability.
The involvement of age-induced genetic/epigenetic alterations in BM niche cells and immunological stressors in hematopoiesis is crucial for understanding the development of age-related hematological malignancies. This comprehensive review provides new insights into the complex interplay between niche cells and HSCs, emphasizing the potential for novel therapeutic approaches that target niche cell functionality and resilience to improve hematopoietic outcomes in the context of aging and metabolic disorders.
Novelty statement
This review introduces novel concepts regarding the plasticity and adaptability of BM niche cells in response to immunological stressors and epigenetics. It proposes that targeted therapeutic strategies aimed at enhancing niche cell resilience could mitigate the adverse effects of aging, diabetes, and obesity on hematopoiesis and clonal hematopoiesis. Additionally, the review suggests that understanding the precise temporal and spatial dynamics of niche-HSC interactions and epigenetics influence may lead to innovative treatments for age-related hematological malignancies.
Graphical abstract
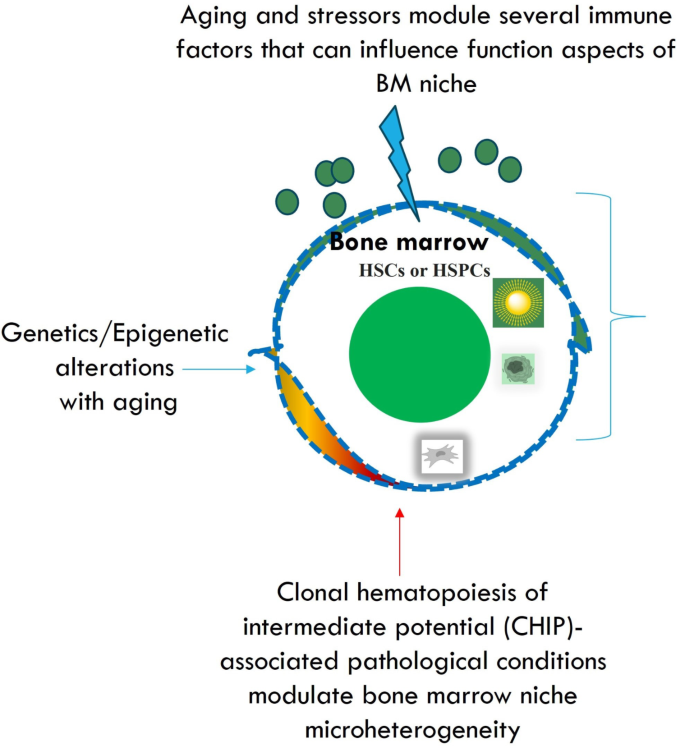
Introduction
During the developmental periods, mesenchymal stem cells (MSCs) and other stem cells exhibit microheterogeneity, specific series of temporal events [ 1 ]. The significant intricate potential of these cells in tissue repair, and cell replacement makes remarkable development of stem cell-based treatments, which require substantial preclinical and clinical studies. However, the unproven efficacy of these cells to ameliorate patient maladies successfully is questionable in terms of science and medical applications.
The bone marrow is a complex and vital component of the human body. It has a diverse and dynamic environment consisting of characteristic cells that work in unison to produce blood and immune cells. The bone marrow houses hematopoietic stem cells (HSCs) and multipotent progenitors (MPPs). Bone marrow niche is perpetually dynamic, where billions of cells are produced to replace transient cells. These are periodically cleared and create an endless supply of blood factors and cells necessary for oxygenation and homeostatic regulation. Bone marrow possesses a unique “demand-adapted” hematopoiesis which is triggered during injury or infection, where there is a surge in production and levels of high-demand cells. This sheds light on the inflammatory response pathway of bone marrow [ 2 ]. The bone marrow (BM) microenvironment is highly vascularized and composed of several niche components such as stromal and nonstromal cells. Niche components inside the BM could act directly on the hematopoietic stem cells (HSCs). For instance, the perivascular cells are confined to the BM and exemplified by the expression of Nestin + mesenchymal stem cells (MSCs). These cells can be categorized into Nestin-GFP bright and Nestin-GFP dim in Nestin-GFP transgenic mice. The Nestin-GFP dim cells are confined to the sinusoids whereas the Nestin-GFP bright cells are confined to the arterioles [ 3 , 4 ]. Stromal cells are categorized into NG2 + cells CXCL-12 abundant reticular cells [ 5 ], and leptin receptor cells [ 6 , 7 , 8 ]. Stem cells confined to the BM could undergo self-renewal and generate a progeny of lineages such as myeloid and lymphoid cells. Mainly, HSCs maintain contact with the osteoblasts in the sinusoidal endothelium in the BM microenvironment. Several anchoring cells are evident in the BM to foster HSCs’ survival, retention, and proliferation. Specifically, endothelial cells (ECs) and MSCs are supporting cells that can promote the survival of HSCs and subsequently foster BM regeneration. Furthermore, sympathetic and parasympathetic innervations of the autonomic system onto the BM could affect the hematopoietic system.
Aging [ 9 ], diabetes [ 10 , 11 ], obesity-induced proinflammatory states [ 12 ], and the formation of fatty BM [ 12 ] are a few potential reasons for clonal hematopoiesis of intermediate potential (CHIP)-associated pathological conditions [ 12 ]. In this review, we have discussed the updated mechanistic role of several niche cells in the BM in the promotion of HSCs proliferation and differentiation upon aging-induced genetic/epigenetic changes, and metabolic diseases in order to target age-related haematological malignancies. We discussed the role of several biological and pharmacological molecules in modulating the HSPCs trafficking in both hematopoiesis during aging-related hematological malignancies by modulating several signaling pathways.
Literature search
We conducted a comprehensive literature review by gathering data from various databases, including Pubmed, Medline, eMedicine, Scopus, Google Scholar, the National Library of Medicine (NLM), and ReleMed. We focused on published reports and articles spanning from 1950 to 2023, exploring studies related to the implications of niche cells within the bone marrow (BM) on hematopoiesis, the influence of neural signaling on hematopoiesis during hematological malignancies while addressing immunological stressors, and their potential clinical implications. Key search terms such as “aging,” “obesity,” “diabetes,” “hematopoiesis,” “BM niche cells,” “epigenetics/genetics”, “clonal hematopoiesis,” “clonal hematopoiesis of indeterminate potential (CHIP),” “immune stressors,” and “hematological malignancies” were utilized to ensure effective literature collection. Relevant articles focusing on the implications of BM niche cells on hematopoiesis during hematological malignancies, particularly in the context of overcoming immunological stressors, were identified for analysis.
BM microenvironment
The bone marrow microenvironment is composed of several different kinds of cell types including hematopoietic stem cell progenitors, osteoblasts, immune cells, osteoclasts, and perivascular cells [ 13 , 14 ]. Primitive hematopoietic cells undergo trafficking to the specific vascular regions of the bone marrow where CXCL12 and E-selectin are abundant. Different kinds of MSCs can regulate the HSCs’ survival in the specialized bone niches. However, the significant diversity and the lineage relationships are yet to be explored vividly for MSCs in the BM. The majority of them are confined across the perivascular space arterioles, and sinusoids and generate several significant niche factors such as SCF and CXCL-12. Consequently, these factors are identified by the leptin receptor [ 15 , 16 ], Nestin [ 17 ], or NG2 (Cspg4) [ 3 ], these were explored by studying the Lepr-cre and Nes-GFP reporter non-inducible mouse lines respectively [ 17 , 18 ]. Bone marrow damage is evident in chemotherapy or radiotherapy after irradiation. Irradiation could damage sinusoids across the bone marrow [ 13 , 19 , 20 ] and the arteriolar blood vessels are preserved in the endosteum. However, it is yet to be examined the role of these factors such as SCF and CXCL-12 in producing either distinct or overlapping cell lineages during hematopoiesis across BM.
- Hematopoiesis
HSCs are multipotent primitive cells that can differentiate into different kinds of blood cells such as myeloid-lineage and lymphoid-lineage cells [ 21 ]. These cells can be abundant in peripheral blood, and bone marrow. These lineages are significantly generated through the self-renewing multipotent HSCs. A vivid understating of the self-renewal and differentiation mechanisms could have significant clinical implications pertinent to disease type and severity. The minimal amount of this HSC population can foster hematopoiesis [ 22 ]. Murine models : Previous studies described the long-term dormancy and quiescence of the HSCs is mediated by several genetic and epigenetic factors as described in the studies related to p21cip1/waf1 by using p21-/- mice [ 23 , 24 , 25 ]. HSCs confined to the bone marrow are significantly involved in the generation of all kinds of blood cells. Albeit HSCs undergo division infrequently, it is suggested that the HSC pool turns over randomly depending on the internal cues and they often undergo division through entering/exiting the cell cycle.
Previous studies have delineated the flow cytometry-based label-retaining assays using BrdU and histone H2B-GFP to observe a specific population of dormant HSCs in murine models within linSca1 + cKit + CD150 + CD48CD34 population [ 26 ]. In addition, computational models described that the dormant HSCs undergo division every 145 days and 5 times in their lifetime [ 26 ]. Stimulation through G-CSF activity or bone marrow injury could induce self-renewal HSCs suggesting their efficacy to switch from dormancy to self-renewal at the time of hematopoietic stress [ 26 ]. As soon as they re-established the homeostasis, they can return to dormancy suggesting that HSCs are not stochastically undergoing the cell cycle but undergo a reversible switch from dormancy to self-renewal at the time of hematopoietic stress [ 26 ].
Several transplantation studies described that the HSCs can live longer when compared to the donor from which they specifically isolated [ 27 ]. Similar to stem cells, telomerase in the HSCs is significantly involved in the stability of chromosomes at the time of cell division [ 28 ]. Furthermore, the HSCs of aged donors are significantly different from young donors [ 29 , 30 ]. For instance, HSCs of aged donors exhibit significantly a rapid cell cycle, altered cell surface phenotypic immune markers, and generate a higher number of myeloid cells, and these HSCs are associated with minimal efficacy in homing to bone marrow when compared to younger individuals [ 31 , 32 , 33 , 34 , 35 , 36 ]. During aged conditions, the HSCs exhibit comparatively a minimal self-renewal capacity when compared to their younger counterparts due to the DNA damage in HSCs [ 37 , 38 ]. However, future studies are required to explore the age-related changes in the behavior of HSCs. Different subsets of HSCs exhibit distinct characteristics such as self-renewal capacities, repopulation kinetics, and differentiation capacities [ 39 , 40 , 41 , 42 ]. The clonal analysis described the HSC compartment in aged individuals and observed the three kinds of HSC subsets reported in younger mice [ 39 , 43 , 44 ]. Normally, the generation of myeloid and lymphoid cells is in a similar ratio to the HSCs self-renewal process in the blood of unmanipulated mice. Myeloid-biased HSCs could produce a minimal number of subsequent generations of lymphoid progeny whereas the lymphoid-biased HSCs could produce a minimal number of myeloid cells [ 39 ]. A minimal number of prethymic T-cell precursors as well as B-cell precursors are produced by the myeloid-biased HSCs. Significantly a slow response of lymphoid progeny has been observed to the IL-7 lymphokine at the time of minimal lymphopoiesis. Myeloid-biased HSCs from young murine models suggested a delay in the onset of repopulation after transplantation but could be conducive to profoundly longer peripheral hematopoiesis when compared to the other kinds of HSCs [ 39 , 45 ].
MSCs derived from the bone marrow are considered rare hematopoietic cells with self-renewal capacity to undergo differentiation into bone, cartilage, and fat. These cells could surround the arterioles and form wrapping loosely around the sinusoidal vessels. Furthermore, along with HSCs, the MSCs can form the CFC-Fs or mesenchymal spheres in in vitro conditions. During the transplantation of MSCs, they could be attributed to the ectopic formation of the hematopoietic niche consequently we can observe BM stromal cells and active haematopoiesis [ 46 ].
Murine models
Human bone marrow-derived CD146 + mesenchymal stem and progenitor cells (MSPCs) upon transplantation through subcutaneous route into the murine models have induced the generation of heterotopic hematopoietic niche associated with host-derived HSCs as well as donor-derived perivascular CD146 + MSPCs [ 47 ]. In addition, the transplantation of CD105 + CD15 + MSPCs obtained from the bone tissue of fetal mice into the renal capsule of adult murine models could foster the formation of ectopic hematopoietic marrow [ 48 ]. Thus, the intricate role of MSPCs requires substantial studies in the irradiated murine models to explore their ability to organize the HSC niche [ 49 ]. MSCs confined to the perivascular region constituted a significant fraction of CD146 in humans [ 47 ] and CXCL12-GFP, Nestin-GFP, leptin receptor, Prx-1-Cre, inducible Mx-1Cre in the murine models could produce the osteoblast cells and foster the generation of several factors to induce the maintenance of HSCs [ 50 ]. The CXCL12 abundant reticular (CAR) cells are confined to the sinusoids and they were co-localized with HSCs across bone marrow [ 51 ]. For instance, irradiating the mice with CXCL-12 expressing BM cells resulted in the depletion of HSCs consequently causing defects in both adipogenic as well as osteogenic efficacy of BM [ 5 ]. Furthermore, the human CD146 + skeletal stem cells can undergo localization adjacent to sinusoids across BM and are involved in fostering substantial amounts of HSC niche factors including SCF, and CXCL-12 [ 47 ]. BM stromal cells express the fibroblast activation protein (FAP) and also a phenotypic expression of CXCL-12, SCF, and Sca-1 is abundant in these cells, and involved in modulating the hematopoiesis [ 52 , 53 ]. For instance, the ablation of BM stromal cells expressing FAP + could cause depression in the BM followed by the eventual occurrence of anemia, hypocellularity, and decline in the osteogenic cells [ 54 , 55 ]. Future studies are warranted to explore these mechanisms in the BM microenvironment.
Endothelial cells (ECs)
The endothelial cells are confined to the perivascular HSC niche [ 56 ] and evidence described the deletion of gp130 cytokine receptors in ECs that could cause hypocellularity of BM and the decline in overall HSCs [ 57 ]. Loss of VEGF receptor-2 functions in the murine models by irradiation caused the impairment of regeneration of sinusoidal ECs consequently blocking the LSK stem/progenitor cells and spleen colony-forming cells [ 20 ]. The ECs could promote the maintenance of HSC in the culture conditions [ 58 , 59 ]. E-selectin expressed by ECs is involved in the HSCs maintenance in BM and for instance, blocking the activity of E-selectin could cause a higher quiescence of HSCs and make them more resistant to irradiated ablation [ 60 ]. These studies described the crucial functions of ECs in the maintenance of HSC niche but future studies required to explore the role of ECs in regulating the HSCs maintenance in the in vivo conditions. It is crucial to explore the activity of ECs involvement in modulating the vascular permeability in NPY-treated mouse models by examining the HSPC egress.
T reg cell’s role in hematopoiesis
T Regulatory cells (Foxp3 + regulatory T cells) play a crucial role in maintaining the immune system and self-tolerance. These specialized cells, characterized by the expression of transcription factor Foxp3, have been extensively studied for their ability to regulate immune response. However, a gray area in research has been the role of T cells in hematopoiesis and post-transplant reconstitution [ 61 ]. In recent times, several experiments have been undertaken to explore the impact of Treg cells on B-cell lymphopoiesis ( Fig. 1 ) and the function of the BM microenvironment [ 61 ]. Researchers have analyzed several T-cell-depleted mice models to understand the relation between T cells and HSC [ 61 ]. In T-cell-depleted mice models, it was evident that the B cell lymphopoiesis was downregulated in the bone marrow, however, the B cell population was replenished in a compensatory manner by the transfer of affected HSCs or bone marrow cells into T-reg competent recipients [ 61 ] (Fig. 2 ). Intriguingly, B-cell reconstitution was not impaired in both syngeneic and allogeneic transplantation models with Treg-depleted mice as recipients [ 61 ].
To further understand the underlying mechanisms, researchers investigated the production of interleukin-7 (IL-7), a growth factor crucial for B-cell lymphopoiesis. It was revealed that the T cell governs the physiological production of IL-7 which is majorly assisted by a subgroup of ICAM1 + perivascular stromal cells [ 61 ]. In the event of T cell depletion, IL-7 production by these stromal cells decreased, suggesting a strong physiological influence of T cells on the differentiation of B cells. There exists a crucial, intricate relationship between T reg cells, B cell differentiation, and the production of essential growth factors. IL-7 can modulate cell fate decisions by increasing the expression profiles of BACH2, EBF1, and PAX5 that concomitantly together conferring to the specification or commitment of B cell progenitors. Previous studies described the significant role of HSC transplantation in patients with T-B + SCID and subsequently concluded the functional role of IL-7 receptor signaling in leukemogenesis [ 62 ]. Understanding these mechanisms can help to gain valuable insights into T cell biology, physiology, and its implications for clinical applications for modulating the immunological homeostasis among normal individuals and individuals with BM transplants [ 61 ].
The impact of Treg cells on immune reconstitution after transplantation is of particular interest. Development of tolerance by T cells is observed in several transplantation models [ 63 ]. Co-infusion of donor Treg cells has been shown to ameliorate graft failure in the allogeneic transplants performed in mice models with induced T cell depletion. Treg cells can provide an immune niche to HSCs, helping them to evade host immunity and favoring their survival. Impaired downstream signaling of inflammatory cytokines such as IFN or TNF can confer to dysregulation of HSCs in the BM [ 64 , 65 ]. These mediators may be crucial in T cell-depleted transplant models. Interestingly, the functional HSC population remained relatively stable in the T-cell-depleted mice model despite the transition of phenotypic HSC into the cell cycle and expansion phase model. However, as a result of T cell depletion, the differentiation of B cells was observed. This indicates that the BM microenvironment aids the B cell differentiation, which was extremely vulnerable to T cell depletion-mediated immune cell activation. This evidence corroborates the role of T cells in regulating the secretion of inflammatory cytokines (which in turn governs HSC quiescence) and BM physiology and function. The HSC and bone marrow environment have susceptibility to host immune cells. The absence of host Treg cells led to increased host-versus-graft BM alloreactivity, resulting in bone marrow aplasia and decreased survival rates. Furthermore, studies suggest that transplant failure is often observed as a result of T cell depletion and has evident manifestations when those mice are subjected to allogenic transplants.
The HSC niche is composed of various essential components, including a cytokine pool which plays a crucial role in HSC progenitor differentiation into lymphoid cell lineages [ 66 ]. Perivascular stromal cells, a crucial component of the HSC niche, secrete growth factors such as CXCL12 and IL-7 for B cell differentiation. In vivo T cell-depleted mice models successfully restored donor B-cell reconstitution when administered external IL-7, highlighting that T cells govern IL-7 production. Subsequently, IL-7 has also been used to restore immune response post-transplant in preclinical studies and clinical trials [ 67 , 68 ].
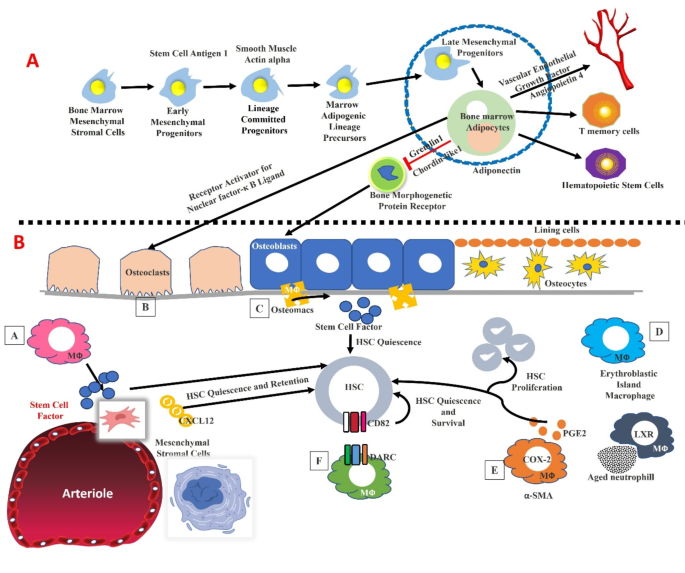
( A ). Bone marrow adipocyte lineages and their role in the hematopoietic stem cells and their role on the osteoblasts, and osteocytes across the bone marrow. ( B ). Heterogeneity of macrophages inside the bone marrow and their role in the hematopoiesis by modulating the functional aspects of niche cells. HSCs dormancy and their maintenance have relied on the macrophages. For instance, the MSCs are modulated by the signals induced by the macrophages. In addition, the macrophages are associated with erythroid progenitors, and factors such as alpha-SMA, DARC, and LXR are expressed by macrophages which could significantly modulate the hematopoiesis by altering HSC function
Administration of IL-7 to syngeneic transplanted mice with adequate T cell levels showed no improvement in the immune reconstitution when compared to their untreated counterparts which proves the satisfactory levels of IL-7 observed in the model and low levels of IL-7 observed only in T cell-depleted mice models [ 61 ].
Osteoblast role in hematopoiesis
Recent studies have shed light on the individual contributions of the different hematopoietic lineages, and the evidence thus far suggests that osteoblasts and vasculature are particularly important players in this intricate multiple lineage system [ 69 , 70 , 71 ]. CD146 + subendothelial cells have been identified as skeletal progenitors that possess an innate ability to restore and reorganize the hematopoietic niche by creating a conducive environment for transplantation in humans [ 47 ]. Studies show that the external endosteal surface has abundant vasculature, whose walls exist in close contact with osteoblasts [ 13 , 19 ].
The trabecular bone lacks endosteal and perivascular/vascular niches, although osteoblasts and vascular cells have different functions. Several reports described the role of osteoblasts within the BM, especially their contribution to maintaining and stabilizing the HSC niche when compared to the osteoblast precursors in different stages which could aid the development of B lymphocytes, which is one of the well-elucidated specific hematopoietic lineages. These studies could provide significant implications for therapeutic interventions, such as BM transplantation and regenerative medicine [ 72 ]. Anatomic evidence suggests a longstanding supportive role of osteoblasts in the hematopoiesis within the bone marrow [ 73 , 74 ]. Osteoblasts do not directly influence HSC function and their maintenance; however, they do govern B cell lineage progenitor activity. This is evident in experiments where osteoblast ablation in adult mice led to the destruction of common lymphoid progenitors (CLPs) whereas B-cell lymphopoiesis was enhanced in cultures enriched for osteoblasts. Cultures enriched for osteoblasts support B lymphopoiesis and ablation of osteoblasts in adult mice acutely depletes common lymphoid progenitors (CLPs) [ 75 , 76 ]. Gs-alpha knockout osteoblasts are essential for PTH receptor signaling and dramatically reduce pro and pre-B cells which are restored with exogenous IL-7 [ 77 ]. Nearly 30% of IL7R + Lineage-bone marrow cells enriched for early lymphoid progenitors are recruited instantaneously towards bone lining cells at the endosteum [ 15 ] (Fig. 1 ).
Macrophages
Macrophages localized in the bone marrow niche are major cell populations that facilitate hematopoiesis by modulating the role of downstream HSCs and MPPs. Macrophages possess an exceptional sensing capacity towards inflammatory markers and they can adjust to the dynamic environment while reducing and/or promoting inflammation. They have a significant impact on HSC function. Therefore, macrophages have a profound impact on hematopoiesis and can be a significant therapeutic target where macrophages are involved in disease pathogenesis. CXCL12 is a chemokine factor that enhances the proliferation and maintenance of B lineage progenitors [ 78 , 79 ] and CLPs [ 80 , 81 ]. One of the fundamental processes that macrophages are involved in is the clearance of apoptotic cells through a process known as efferocytosis. This process is essential for the efficient removal of dead or damaged cells. Macrophages facilitate efferocytosis by efficient disposal of neutrophils and activation of the LXR-dependant mechanism. This substantiates the influence of macrophage physiology and function on the homeostatic regulation of blood progenitors. Any dysfunction in circadian rhythms due to aging comorbid with obesity and lifestyle and environmental stress can have profound consequences on the mobilization of HSC and HSPC, which has to be explored through substantial future studies. Inflammatory signaling facilitates HSC and HSPC mobilization into circulation simultaneously with the production and release of granulocytes [ 82 , 83 , 84 ]. The driving factor for mobilization and granulopoiesis is the G-CSF, a growth factor that facilitates proliferation and differentiation [ 85 , 86 , 87 ]. The consequence of this event is the downregulation of CXCL12, G-CSF receptor signaling is often not necessary in hematopoietic progenitors themselves [ 88 ] ( Fig. 1 ) , but macrophages are typically required for the G-CSF-dependent HSC mobilization [ 89 ]. G-CSF can induce a dramatic reduction of macrophage levels in the bone marrow but the levels remain relatively unaffected in other tissues like the spleen [ 90 , 91 ]. This demonstrates that localized reduction of the macrophage population inside the bone marrow could foster the mobilization of HSC. This was consistently observed in a study involving “clodronate-loaded liposome” mediated depletion of macrophages which resulted in HSC mobilization and concomitantly promoted the G-CSF-driven HSC mobilization [ 92 ].
The resulting mobilization under the above circumstances accounts for a high number of HSCs in the circulation which suppresses CXCL12, and various HSC retention signals [ 92 , 93 ], some of which may drive the HSC/HSPC pool to the dormancy [ 94 ]. Furthermore, macrophages are a diverse population of cells that play crucial roles in tissue homeostasis, inflammation, and immune responses during aging, and obesity. Along with the mobilization of HSCs, macrophages are required for the HSCs bone marrow engraftment post-transplant [ 94 ].
Bone marrow macrophages exhibited radioresistance after irradiation and this resistance persisted for 30 days post-radiation. These tolerant macrophages were typically significant for HSC engraftment and reconstitution post-radiation, which is evident by the ‘reduction of HSC engraftment’ by CD169 expressing macrophage depletion mediated by diphtheria toxin receptor (DTR) [ 94 ]. This substantiates the persistence of macrophages post-lethal radiation across BM, as these macrophages can undergo slow clearance or proliferation and also possess self-renewal capacity potentiated by M-CSF. Exogenous M-CSF facilitates the expansion of the host macrophage pool which is demonstrated to have a protective effect on native macrophages and reduction of graft vs. host disease during transplantation [ 94 ].
In an independent study, HSC retention and mobilization in bone was potentiated by the depletion of macrophages which consequently resulted in stable hematopoietic chimerism [ 95 ]. However, another study provided contrasting evidence that the reduction in HSC engraftment due to macrophage depletion consequently when combined with radiation has led to the development of a proinflammatory environment which resulted in impairment of engraftment [ 96 ]. Clod-lip-induced macrophage depletion is not the sole factor for inflammatory response. This inconsistency highlights the important gaps in the interpretation of macrophage depletion studies since they only allow for in vivo modulation of inflammation and immune cells. Clod-lip-mediated macrophage depletion has been widely studied and the underlying mechanism is well established. It essentially involves apoptosis of the phagocytic cell (engulfing liposome) which is triggered by the release of the bisphosphonate intracellularly [ 97 ]. However, its deletion in osteoblastic cells led to a reduction in CLPs and other premature lymphoid progenitors in the BM without affecting HSCs [ 15 ]. Hence some premature lymphoid progenitors rely on the osteoblast microenvironment rather than the HSC-specific niche. Other lineage-specific microenvironments include the erythroid niche where macrophages are crucial for erythropoietic cell maturation [ 98 ]. An in-depth analysis of the erythroid niche is necessary to determine characteristic cellular components to further elucidate the interlink between this niche and existing HSC lymphoid progenitor niches. In vivo, experiments involving induced and conditional alteration or deletion of niche-specific factors and analyzing the manifestations and effects on stem/progenitor cell maintenance provides insights on the stem cell specific-niche and specific progenitor belonging to haematopoietic system, restricted by the precision of available Cre alleles. Thus, gaining an understanding of the cellular and functional differences between these niches is important for advancing our knowledge of haematopoiesis and immune cell development. It opens up new avenues for research into how the bone microenvironment influences the fate and function of different types of blood cells. This knowledge could have implications for developing new strategies to manipulate immune cell production and function, potentially leading to novel therapies for immune-related disorders.
Perivascular cells
Perivascular cells are a crucial niche for modulating the role of adult HSCs. These perivascular cells coexist in an interactive environment with HSCs in adult BM and serve as precursors to mesenchymal stem/stromal cells (MSCs) that foster differentiation into various cell kinds [ 99 ], including osteoblasts, adipocytes, and chondrocytes. Pericytes are typically located on the abluminal side of blood vessels; they also maintain significant association with endothelial cells (ECs) and express markers including CD146, NG2, PDGFRβ, α-SMA, Nes, and LepR [ 99 ].
However, pericytes are not a homogenous population. Different subsets exhibit distinct immunophenotypes and mesenchymal features, including varying capacities to support hematopoiesis. Both arteriolar and sinusoidal pericytes play crucial roles in regulating HSC behavior, maintenance, and HSC quiescence, and trafficking inside the adult BM via paracrine signaling [ 99 ]. Various studies have described hematopoietic-supportive pericyte subpopulations with the aid of diverse markers through murine experiments. Arteriolar pericytes, are marked by upregulated α-SMA and NG2 levels, and they are associated with the maintenance of HSC quiescence and long-term repopulating capacity. Sinusoidal pericytes, often expressing CD146 and PDGFRβ, are implicated in the regulation of HSC mobilization and homing [ 99 ]. For instance, Nestin-expressing pericytes have been shown to secrete high levels of CXCL12, a critical chemokine for HSC retention in the BM niche. Similarly, LepR-positive pericytes have been identified as key regulators of HSC maintenance through their support of the sinusoidal microenvironment. These findings suggest that specific pericyte subpopulations create distinct niche environments that differentially influence HSC function [ 99 ]. Detailed molecular profiling of pericyte subpopulations will enhance our understanding of their specific roles in HSC regulation. Single-cell RNA sequencing could provide insights into the gene expression profiles and signaling pathways active in different pericyte subsets [ 99 ]. Investigating the functional impact of pericyte-HSC interactions using advanced in vivo models and genetic manipulation techniques will clarify the mechanisms through which pericytes influence HSC behavior. Conditional knockout models targeting specific pericyte markers or signaling pathways could elucidate their roles in HSC maintenance and mobilization. Leveraging the knowledge of pericyte subpopulations to engineer BM niches in vitro could have significant implications for HSC transplantation and regenerative medicine. Creating bioengineered niches that mimic the in vivo perivascular environment may improve the expansion and functional maintenance of HSCs ex vivo. Exploring the changes in pericyte function and HSC support with aging or in disease contexts, such as myelodysplastic syndromes or leukemia, will provide insights into how pericyte-HSC interactions are altered in pathological states. This could lead to targeted interventions that restore normal niche function and improve patient outcomes [ 99 ].
The rapid growth of the skeletal system during embryonic and postnatal life necessitates a coordinated interplay between cell proliferation, differentiation, mineralization, and the expansion of local vasculature [ 100 , 101 ]. Several bone-forming cells including chondrocytes and osteoblasts are implicated in this process by releasing VEGF, which stimulates angiogenesis by stimulating VEGF receptors confined on ECs [ 100 , 101 , 102 ]. Vascular ECs can generate paracrine signaling which could modulate growth as well as regeneration in multiple organs including the skeletal system [ 103 , 104 , 105 , 106 , 107 ]. For instance, osteogenesis is particularly mediated through a specialized capillary EC subtype known as type H; type H-containing capillary ECs are characterized by the expression of CD31/PECAM1 as well as Endomucin (CD31 hi Emcn hi ) markers. These cells are predominantly located in the metaphysis and endosteum of postnatal long bones [ 103 , 104 , 105 , 106 , 107 , 108 ]. Type H ECs not only promote angiogenic growth but also release molecular signals that act on osteoprogenitor cells, subsequently coupling angiogenesis with osteogenesis. Conversely, type L ECs, which exhibit lower CD31 and Emcn expression (CD31 lo Emcn lo ), together associated with sinusoidal vessel network across BM [ 103 , 104 , 105 , 106 , 107 , 108 , 109 ].
Future studies are required to explore research into modulating the VEGF signaling pathway specifically in type H ECs might offer novel approaches to enhance bone healing and repair, particularly in aging populations where bone regeneration is impaired. In addition, identifying and validating biomarkers for type H and type L ECs could facilitate the monitoring of therapeutic outcomes and the development of precision medicine approaches for bone-related diseases. By addressing these future directions, it is possible to develop more effective treatments for bone diseases, enhance bone repair, and improve overall skeletal health [ 109 ].
Correlation of arterial and sinusoidal niches in promoting HSC quiescence
Cell cycle quiescence is significant to maintain HSC’s role in BM. Although several stromal cells are proposed as HSC niches, the precise spatial localization of HSCs in the quiescent stage yet remained ambiguous. A study by Yuya Kunisaki et al. 2013 described that quiescent HSCs are typically confined to small arterioles located across endosteal BM. These arterioles are positively ensheathed for NG2 + pericytes which are distinct from LepR + cells. Decline in NG2 + cells could cause enhanced HSC cycling and a decline in the repopulating HSCs on a long-term basis suggesting that arteriolar niches are crucial to maintaining HSC quiescence [ 3 ].
Structurally, arterioles and sinusoids are different; these structural differences are reflected in the altered unique transcriptional programs of ECs across arterioles and venules. For instance, arteriolar Nes peri cells and sinusoidal Nes retic cells are uniquely differentiated through their transcriptional activities; for instance, Nes peri cells exhibit a greater enrichment in genes related to cell cycle quiescence as well as for maintaining the HSC niche. Under genotoxic stress, mitotically active Nes retic sinusoidal niche cells are undergoing destruction but quiescent Nes peri arteriolar niche cells exhibit chemoresistance. This dual association of hematopoietic and mesenchymal stem cells with arterioles suggests that these vessels could coordinate hematopoietic and regeneration of stroma [ 3 , 110 ]. Further investigations have revealed that the interaction between HSCs and their niche is more complex than previously understood. HSCs in periarteriolar niches are mediated in a quiescence state through the influence of NG2 + pericytes, which secrete factors that suppress cell cycling. This is contrasted by the LEPR + perisinusoidal niche, which supports more active HSC states. The dynamics between these niches suggest a sophisticated regulatory mechanism that ensures the preservation and timely activation of HSCs in response to physiological demands. Moreover, the chemoresistance observed in quiescent Nes peri arteriolar niche cells under genotoxic stress highlights a potential therapeutic target for enhancing HSC protection during chemotherapy. Understanding the molecular signals that confer this resistance could lead to strategies to bolster HSC resilience in patients undergoing aggressive treatments. Detailed molecular studies to elucidate the specific signals and pathways involved in maintaining HSC quiescence within arteriolar niches enable the development of therapeutic interventions that can mimic the protective environment of the arteriolar niche to enhance HSC survival during treatments like chemotherapy. Investigating the interactions between different niche cells, including pericytes, ECs, and mesenchymal progenitors is essential to develop a comprehensive map of HSC regulatory networks. By addressing these, it is possible to understand HSC regulation and develop novel therapeutic approaches to enhance hematopoietic health and regeneration [ 3 ].
Bone marrow adipocytes (BMA) are metabolically active and possess abundant lipid reserves, mitochondria, and endoplasmic reticulum. The number of bone marrow adipocytes is variable during growth and development as is influenced by various internal and external factors like osteoporosis, aging, and calorie-deficit diets [ 111 ]. Current research indicates that bone marrow adipocytes have an inhibitory effect on hematopoiesis [ 111 , 112 ]. Caudal vertebrae are composed of adipocytes, a low number of HSCs, and short-term progenitors when compared to the thoracic vertebrae which contain no adipocytes. Moreover, the pharmacological and genetic downregulation of adipogenesis expedites the restoration of hematopoiesis post-irradiation and bone marrow transplant [ 112 ]. However, there is no concrete evidence to justify the role and influence of BMAs on HSC and the bone marrow niche. Recent evidence suggests that adipocytes localized in long bones aid restoration of hematopoiesis post-irradiation by providing a crucial HSC survival factor, stem cell factor (SCF) [ 113 ]. However, adipocytes localized in tail vertebrates are known to downregulate hematopoiesis [ 114 ]. Fatless A-ZIP/F1 mice models when exposed to radiation resulted in the loss of bone marrow cellularity and HSC inside the long bones, but a higher number of HSCs was observed in caudal vertebrae [ 114 ]. This stark contrast was associated with the increased vasculature in the tail vertebrae of the mice, which is absent in long bones [ 114 ]. The main requirement for restoration of hematopoiesis is an adequate vascularization of bone marrow [ 20 ]. Along with SCF, adiponectin and leptin are two other biomolecules produced by adipocytes that could enhance the proliferation of HSC [ 115 , 116 ].
The rhesus macaque model has been used to validate the relation between BMAs and hematopoiesis in primates. HSPCs reside adjacent to BMAs. Additionally, BMAT (Bone marrow adipocyte tissue)-enriched medium facilitates the HSC proliferation and differentiation ex vivo. A quantitative protein examination of the BMAT-conditioned medium was performed to understand the underlying mechanism. Out of a total of 994 BMAT-derived proteins, including TGFB1, FBLN1, IGFBP2, LGALS1, TIMP1, and C3, were reported to possess the capacity to regulate and increase the differentiation, adhesion, and mobility of HPSC [ 117 ]. Of the 994, 430 proteins have a complex framework, possess paracrine function, and have origin from microvesicles or exosomes. It is also essential to understand that BMAT contains cells apart from BMA including macrophages and granulocytes [ 117 ]. Thus, the proteins derived from the BMAT could play a regulatory role in HSC function via these immune cells. BMA’s role in leukemia is a subject of debate and is largely lineage-specific. In vitro and in vivo experiments provide evidence that BMAs inhibit T-acute lymphoblastic leukemia (ALL) proliferation in ALL conditions [ 118 ].
Further, BMAs, when cocultured with AML blasts, exhibited typically diminished apoptotic effect and higher proliferative effect in acute myeloid leukemia (AML) [ 119 ]. AML blasts enhance the lipolysis of BMAs and the resulting fatty acids are transported to AML cells where they undergo β-oxidation. Recent evidence suggests that AML reduces the adipocyte cellularity in the bone marrow and AML xenograft implying that AML selectively influences adipocyte number along with potentiating lipolysis of existing adipocytes [ 120 ]. A comprehensive analysis of the global transcriptome of BMSCs isolated from AML or healthy patients reported altered adipogenic differentiation caused by AML [ 120 ]. Furthermore, transwell assays were conducted to explore the interlink between BMA reduction and mitigated myelo-erythropoiesis in AML conditions. From this study, it is evident that BMAs can promote the maturation of myeloid and erythropoietic cells. In another study, adipogenesis was activated by GW1929, a PPARγ agonist, which was found to restore hematopoietic maturation in addition to the inhibition of leukemic growth [ 120 ].
In conclusion, BMAs are crucial for the normal maturation of myeloid and erythropoietic cells and hence a valid target for therapy to ameliorate bone marrow failure observed in AML (Fig. 1 ). BMAs are susceptible to changes in homeostatic metabolism and hence need to be researched further to accurately determine their physiology and role in the pathogenesis of diseases like hematological malignancies [ 2 , 121 ].
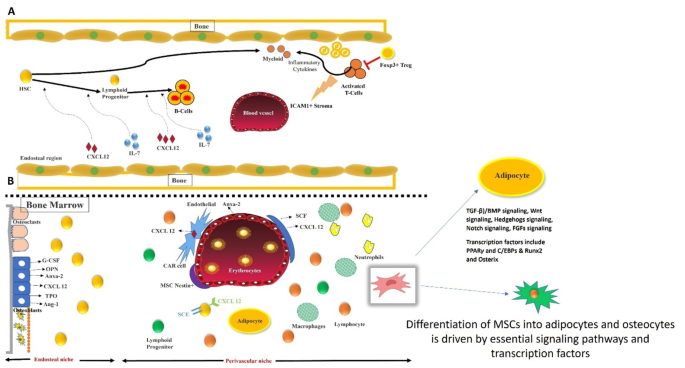
The fine balance between adipogenic and osteogenic differentiation of MSCs is orchestrated by crucial signaling pathways and transcription factors. Signaling pathways such as TGF-β/BMP, Wnt, Hedgehog, Notch, and FGFs play pivotal roles in this regulation. These pathways influence key transcription factors, PPARγ and C/EBPs for adipogenesis, and Runx2 and Osterix for osteogenesis-ensuring a precise differentiation balance. ( A ). The role of regulatory T cells (Tregs) in the bone marrow environment is significant. Foxp3 + Tregs can influence the activity of cytotoxic T cells and ICAM1 + perivascular cells. Activation of T cells leads to the depletion of Tregs, which in turn reduces UL-7 production by perivascular cells, affecting B cell differentiation from hematopoietic stem cells (HSCs). ( B ) HSCs within the bone marrow microenvironment are supported by the endosteal and perivascular niches, including osteoclasts, osteoblasts, and other supportive cells. Factors such as G-CSF, osteopontin, annexin-2, thrombopoietin, and angiopoietin-1 regulate HSC functions in lineage commitment. Additionally, osteoblasts, endothelial cells, LepR + perivascular cells, and Nestin + MSCs producing CXCL12 and CAR cells play crucial roles in the homing, self-renewal, and differentiation of HSCs, facilitating hematopoiesis
Primitive hematopoietic cells are confined to the endosteal surface while the progenitors are located centrally within the marrow space. Intravital microscopy experiments were conducted along with HSPCs labeled with significant markers have further confirmed the proximity of HSPCs to the endosteal osteoblasts during the HSPC engraftment conditions where a significant number of mature progenitors were positioned further from osteoblasts [ 13 , 19 ]. Furthermore, migration of hematopoiesis from fetal liver to bone marrow greatly relies on normal bone formation and turnover during embryonic development. Previous reports suggested that the mice lacking runx2, a key transcription factor for osteoblast differentiation, developed weak, demineralized, or structurally skeletons [ 122 , 123 , 124 ] which led to compensatory extramedullary [ 124 ].
Mice with defective M-CSF exhibit typically reduced levels of osteoclasts which eventually results in osteopetrosis and extramedullary hematopoiesis [ 125 ]. In vitro studies have demonstrated the supportive function of stromal cells [ 126 ] to the osteoblast lineage to foster hematopoietic cell differentiation [ 127 , 128 ]. Targeted ablation of osteoblasts was carried out in the mice presenting differentiated osteoblasts with herpes simplex thymidine kinase through ganciclovir administration [ 76 ]. This led to a reduction in bone marrow niche and conferred to the extramedullary hematopoiesis, highlighting the role of osteoblasts in supporting hematopoiesis in vivo across the BM [ 129 ]. The osteoblast ablation resulted in a downregulation of B-cell lymphopoiesis and erythropoiesis in the BM, eventually mitigating the primitive hematopoietic cell population in bone marrow [ 75 , 129 ]. Osteoblasts have also been implicated in HSC mobilization, particularly in response to G-CSF [ 130 , 131 ] (Fig. 2 ). However, the precise molecular mechanisms and the requirement for direct contact between HSCs and osteoblasts in vivo are still being investigated.
Parathyroid hormone exerts a synergistic effect through osteoblasts on B lymphopoiesis. PTH/PTH-related peptide receptor (PPR) signaling plays a role in osteoblast-mediated B-cell development. Genetic manipulation or alteration of either osteoblast-specific PPR [ 132 ] or the BMPR1a receptor [ 133 ] results in a higher number of osteoblasts and increased HSC niche [ 133 ]. However, a low level of osteoblasts was observed in biglycan knockout mice which is independent of any hematopoietic defect or decrease in HSC [ 134 ]. This indicates that osteoblast is not necessarily the sole determining factor of the HSC population [ 134 ].
Osteoblasts can regulate functions of various hematopoietic factors including angiopoietin-1 [ 135 ], osteopontin [ 136 , 137 ], thrombopoietin [ 138 ], Wnts [ 139 ], and extracellular calcium [ 140 ]. A recent study suggested that the overexpression of Notch ligand Jagged-1 (Jag1) in osteoblasts by PPR activation directly leads to the increase in the HSC population, thus implicating notch signaling as a factor for hematopoiesis. The overexpression of HSCs can be suppressed by γ-secretase inhibitors [ 132 ]. It has also been demonstrated that the deletion of Jag1 Mx1-Cre-mediated in the microenvironment yielded no phenotype [ 141 ].
The role of N-cadherin is widely debated among researchers, some who advocate its synergism and importance [ 19 , 133 ] and others who contest the same [ 134 ]. N-cadherin associated with β-catenin/Wnt-signaling has a significant role in the HSCs’ interactions with their niche [ 142 ]. Previous studies reported the role of osteoblast N-cadherin mainly cadherin-11 confined to the osteoblasts is upregulated during differentiation. Another study reported that overexpression of c-Myc and Rbm15 can mitigate N-cadherin expression and speculated that the mitigation in the expression of N-cadherin is required for the release from the stem cell niche [ 143 , 144 , 145 ].
Therefore, while the role of osteoblasts in maintaining the homeostasis of the HSC niche is validated through the studies of in vivo models, the crucial underlying mechanisms remain uncertain. The molecular mechanisms underlying the cross-talk between the osteoblast lineage of the skeletal system and perivascular/vascular cells of the hematopoietic niche is another gray area. Cell-specific ablation of factors will be crucial to addressing several of these issues and bridging the research gap [ 72 ].
In vitro cultures of HSCs are not feasible unlike other stem cells sourced from other tissues which can be readily cultured in vitro. This could hinder their therapeutic and transplantation scope, one of them being gene therapy where the transfected HSCs need to be cultured to assess the quality before transplantation. Several factors could influence HSC survival through osteoblasts and the pleiotrophin supplementation promoted HSC survival in vitro [ 156 ] and the lack thereof resulted in HSC reduction and dysregulation of haematopoiesis [ 157 ]. Pleiotrophin is produced by sinusoidal liver endothelial cells and perivascular stromal cells expressing CXCL12 to promote HSC function [ 157 ]. A key slit receptor, Robo4, is expressed by both endothelial cells and HSCs. This receptor governs the HSC localization in bone marrow microenvironment [ 158 , 159 ]. The slit2 ligand exhibits selective activity towards MSCs and some osteoblast lineage cells. This indicates that both pleiotrophin and Robo4/Slit2 are essential components of the perivascular environment. Evidence also suggests a possible positive or negative influence of Tenascin-C [ 160 ], osteopontin [ 136 , 137 ], and non-canonical Wnts on the HSC population (Tables 1 and 2 ).
Importance of the different signaling pathways involved with BM niche
As we discussed above, osteoblasts were the first cell type identified to support HSC expansion in vitro, notably via the presentation of granulocyte G-CSF [ 161 ]. These cells secrete a variety of proteins, including angiopoietin-1, CXCL12, SCF, and TPO, all of which are pivotal for promoting HSC growth [ 136 , 140 , 162 ]. Additionally, osteopontin and SDF-1α produced by osteoblasts are crucial for the mobilization and egress of HSCs [ 163 ]. The perivascular niche, encompassing ECs, perivascular stromal cells, and MSCs, plays an essential role in maintaining HSC physiology near blood vessels. MSCs, which are highly heterogeneous, express markers such as CD146, CXCL12, Nes, and LepR, all of which are vital for HSC survival [ 16 , 47 ].
Primary ECs obtained from non-hematopoietic organs have been shown to enhance HSCs repopulation in vitro [ 164 ]. HSC migration is facilitated by derived from CD31 + ECs as well as E-selectin ligand-1 (ESL-1) in HSPCs. Blocking this interaction results in HSCs becoming quiescent and exerting resistance to the irradiation [ 60 , 165 ]. Various mature cells in the bone marrow also play significant roles in modifying the niche. Trophic endosteal macrophages, for example, support both osteoblast function and the entire endosteal HSC niche. Their absence can lead to HSC egress into the bloodstream [ 93 , 166 ]. Non-myelinating Schwann cells regulate HSC pools through the modulation of TGF-β signaling [ 39 , 166 ]. Expansion of HSPCs is also regulated by the Notch stimulation [ 167 ]. Notch signaling plays a dual role: it could foster the morphology development and artery specification subsequently mediates communication among niche cells by enabling Notch receptor expression or ligands. Another critical signaling is the involvement of the canonical Wnt pathway. For instance, overexpression of β-catenin has been associated with a higher hematopoietic cascade [ 168 ]. Quiescent LT-HSCs are characterized by the Frizzled 8 expression, which antagonizes Wnt signaling, while Wnt5a maintains HSC quiescence through the inhibition of Wnt3a-mediated canonical Wnt pathway [ 133 ]. In addition to these pathways, other signaling mechanisms indirectly influence HSC development, including BMP as well as the Hedgehog pathway. BMP pathway can support the function of spindle-shaped N-cadherin + osteoblasts to control bone marrow niche size and also the fate of HSCs. Sonic Hedgehog is involved in fostering primitive HPCs differentiation and myeloid differentiation [ 169 , 170 ]. Further, in vivo studies are required for conducting comprehensive in vivo studies to better understand the dynamic interactions within the BM niche and their impact on HSC behavior under various physiological and pathological conditions. As part of regenerative medicine studies, it is crucial to explore the potential of using niche-modifying agents in regenerative medicine to enhance bone and vascular health, thereby improving outcomes in BM transplantation and other hematological therapies.
Adipo-osteogenic differentiation of MSCs and molecular signaling
MSCs undergo a 2-step differentiation involving lineage commitment and maturation, transitioning from multipotent stem cells to lineage-specific progenitors and finally into specialized cell types. Key signaling pathways regulating MSC differentiation include TGFβ/bone morphogenic protein (BMP), Wnt, Hedgehog (Hh), Notch, as well as fibroblast growth factors (FGFs) [ 171 ].
The TGFβ/BMP signaling pathway is known to have dual roles in MSC differentiation, affecting both adipogenesis and osteogenesis [ 172 ]. This pathway operates through canonical Smad-dependent and non-canonical Smad-independent mechanisms, such as the p38 MAPK pathway [ 173 ]. Activation of these pathways regulates the expression of key transcription factors like runt-related gene 2 (Runx2/Cbfa1) [ 174 ] and PPARγ, which in turn dictate MSC differentiation [ 172 ]. The cytokine composition in the MSC microenvironment is thus crucial for determining lineage commitment.
Wnt signaling can confer osteogenic differentiation but impair adipogenic differentiation [ 175 ]. For instance, Wnt3a stimulates osteogenesis via TAZ activation by PP1A-mediated dephosphorylation, and YAP/TAZ can modulate Wnt signaling-induced osteogenesis [ 176 , 177 ]. Aging-related enhancement in adipocytes is linked to reduced Wnt10b levels. Furthermore, β-catenin loss in the developing mouse uterus mesenchyme switches differentiation towards adipogenesis [ 178 , 179 , 180 ].
Notch signaling plays a complex role in MSC differentiation. Impairment of Notch pathway promotes autophagy-associated adipogenesis by modulating PTEN-PI3K/AKT/mTOR pathway [ 181 ]. Notch also suppresses osteogenesis by inhibiting Wnt/β-catenin signaling but can promote osteogenesis through BMP2 signaling cross-talk [ 173 , 182 ].
Hedgehog signaling is downregulated during adipogenesis, with decreased Gli expression. Hedgehog pathway actuation inhibits adipogenesis by repressing PPARγ and C/EBPα expression, while Gli inhibition promotes adipogenesis [ 183 ]. Conversely, Hedgehog signaling supports osteogenesis [ 184 , 185 , 186 ] and can interact with BMP signaling to enhance Smad-mediated osteogenesis, highlighting its pro-osteogenic and anti-adipogenic roles [ 171 ] (Fig. 2 ).
Impact of aging on adipo-osteogenic differentiation
Aging is associated with a shift in the differentiation balance of MSCs, leading to increased bone marrow adiposity and decreased osteogenesis [ 187 , 188 , 189 ]. A previous study identified several molecules linked to age-related osteogenic potential loss, including decreased levels of chloride intracellular channel 1 (CLIC1) as well as prohibitin, and increased levels of LIM and SH3 domain protein 1 (LASP1) and annexin V. Furthermore, aging also increases ROS- mediated oxidative stress, which play significant role in age-induced bone loss as well as differentiation balance through pathways involving FOXO, Wnt, or PPARγ [ 189 , 190 , 191 ]. For instance, PPARγ, a key transcription factor to modulate adipogenesis, inhibits osteoblast differentiation. Increased PPARγ expression in aged MSCs promotes adipogenesis and inhibits osteogenesis [ 171 ]. It is crucial to investigate the interactions between different signaling pathways (e.g., TGFβ/BMP, Wnt, Notch, Hedgehog) to better understand the regulatory networks governing MSC differentiation. Subsequently exploring how microenvironmental factors, such as cytokine composition and mechanical stimuli, influence MSC lineage commitment and differentiation is needed. This could lead to targeted therapies that modulate these factors to promote desired differentiation outcomes. By addressing these research directions, we can better harness the potential of MSCs for regenerative medicine, particularly in the context of aging and bone-related diseases [ 171 ].
BM niche regulates stem cell trafficking and age may influence the trafficking in the following signaling pathways
The bone marrow microenvironment is composed of several different kinds of cell types including hematopoietic stem cell progenitors, osteoblasts, immune cells, osteoclasts, and perivascular cells [ 13 , 14 ]. Irradiation could damage sinusoids across the bone marrow [ 13 , 19 , 20 ] and the arteriolar blood vessels are preserved in the endosteum.
Aging is a highly intricate physiological process associated with significant alterations in tissue-specific changes due to alterations in gene expression and cell composition [ 192 ]. In the bone marrow (BM), aging leads to an expansion of the HSC pool, with HSCs exhibiting a biased differentiation toward myeloid progenitors at the expense of lymphoid ones, along with a diminished regenerative potential [ 35 , 193 ]. Mitosis analysis described that HSCs, and MPPs exhibit a quiescent nature in a steady state whereas the granulocyte-macrophage lineage-restricted progenitors (GMLPs) exhibit a higher proliferation rate [ 194 ]. Aging in HSCs is characterized by various intrinsic changes, including loss of cell polarity, heightened Wnt5a non-canonical signaling, disrupted autophagy [ 195 ], deregulation of the mitochondrial unfolded protein response, reduced mitochondrial acetylation mediated by SIRT3, and alterations in the epigenome, including increased symmetry of epigenetic division [ 196 , 197 , 198 , 199 , 200 , 201 , 202 , 203 ]. These intrinsic alterations could affect the functions of HSC function irrespective of the BM niche, a phenomenon termed “intrinsic” HSC aging, extensively reviewed elsewhere [ 204 ]. Recent investigations into middle-aged BM microenvironments have identified decreased IGF1 levels as a crucial aging-promoting factor affecting both HSCs and niche cells. Restoring IGF1 signaling has been shown to rescue Cdc42 and tubulin polarity, reduce γH2AX focus, and alleviate myeloid differentiation skewing in middle-aged LT-HSCs [ 205 ]. Interestingly, fasting-induced decrease of IGF1-dependent stimulation of PKA activity has been identified as a key factor promoting HSC self-renewal, balanced differentiation, stress resistance, and regenerative capacities after chemotherapy in aged mice [ 206 ]. This apparent discrepancy might be explained by the different downstream pathways activated by IGF1, with fasting-induced IGF1-mediated effects passing through PKA activation, while aging-associated effects activate the mTOR signaling [ 205 , 206 ]. This suggests that more research is needed to fully understand the regulation of HSC function by IGF1 during aging. Additionally, aging results in the degeneration and remodeling of various niche compartments, impacting HSC behavior and function on different levels [ 207 ].
As the age increases, the alterations occur in the vasculature, marked by the decline in CD31 high Endomucin −/low arterioles [ 147 , 151 , 208 ]. Stromal cells inside BM also change, such as the depletion of periarteriolar Osteolectin (+) cells, potentially contributing to the decline in lymphoid progenitors [ 209 ]. In contrast, sinusoids remain preserved during aging, potentially explaining the absence of age-related depletion of HSCs in most mouse strains, unlike lymphoid progenitors [ 208 ]. The bone marrow’s inflammatory milieu increases during the aging process subsequently contributing to hematopoietic changes. A higher expression of inflammatory factors, including interferons, IL-1β, IL-6, and TNFα by stromal and hematopoietic cells is evident as the age increases in the individuals fostering increased myelopoiesis [ 4 , 150 , 210 , 211 , 212 ]. Additionally, alterations in nerve fibers within the bone marrow may play a role in age-related changes in hematopoiesis [ 146 , 213 ]. Hence, our study specifically focuses on the influence of neural signaling in the hematopoiesis of aging-related hematological malignancies with subsequent need for the development of gene therapies.
Hematopoietic system during aging
With the global population aging, health implications such as cancer, neurodegenerative, and other several ailments contribute to significant public health concerns [ 214 ]. Aging pertinent to the hematopoietic system disrupts immunity and homeostasis, increasing the risk of blood malignancies due to impaired HSC function [ 35 , 215 , 216 , 217 ]. Myeloid malignancies are more common with age, whereas lymphoid malignancies are more prevalent in younger individuals [ 216 , 218 , 219 ]. Understanding age-related HSC behavior is crucial for addressing the physiology of the hematopoietic system as age increases. Aging in the hematopoietic system manifests through enhanced myelopoiesis, mitigated ability of adaptive immune functions, and decreased HSC functionality, which are essential for sustaining hematopoiesis. The aging process is characterized by alterations in different HSC subset levels, although the regulatory mechanisms, particularly given HSC heterogeneity, are not fully elucidated. A previous report by Tsu-Yi Su et al. shed light on how aging affects different HSC subset functions, marked by CD49b [ 219 ].
Shifts in lineage bias, epigenetic and transcriptional changes with aging
As per the previous reports, both lymphoid-biased as well as myeloid-biased HSC subsets show a shift towards increased myeloid subsets as they age. Additionally, gene expression and regulatory mechanisms in HSCs start to change from the juvenile stage and continue progressively, indicating intrinsic modifications in both cellular and molecular properties due to aging] [ 219 ]. Aging is linked to substantial alteration in the transcriptional as well as epigenetic changes that impact the differentiation of HSCs. Previous reports described differentiation-associated gene loci becoming hypermethylated, while self-renewal-associated loci become hypomethylated, with enhanced histone marks activity in aged HSCs [ 35 , 220 , 221 , 222 ]. The study of epigenetic changes in lineage-biased HSC subsets during aging has faced significant limitations, primarily due to difficulties in separating functionally different HSC subsets. As a result, the specific epigenetic modifications that occur in these lineage-biased HSCs have not been thoroughly investigated. Recent advancements in single-cell epigenomics and improved isolation techniques have begun to shed light on these processes. These innovations allow for a more precise dissection of the epigenetic landscape of HSCs, revealing how age-related changes contribute to lineage bias and functional decline. Understanding these mechanisms is crucial for developing targeted therapies to mitigate age-associated hematopoietic dysfunction.
Tsu-Yi Su et al. [ 219 ]identified integrin CD49b as a significant marker for differentiating functional subsets among primitive Lineage–Sca-1 + c-Kit+ (LSK) CD48–CD34–CD150hi (CD150hi) HSC compartment [ 223 ]. CD49b– HSCs are confined highly to the myeloid-biased cells, whereas CD49b + HSCs predominantly exhibit lymphoid-biased features. Despite transcriptional similarities, these subsets show different profiles of chromatin accessibility, describing epigenetic regulation of these functionally distinct lineage-biased HSCs [ 223 ].
Myeloid shift and HSC composition with aging
The increased myeloid output in aging has been attributed to the mitigated efficacy of HSCs to generate lymphoid subsets. Identification of distinct subsets of HSCs describes alterations in the clonal composition of HSCs which could drive enhanced subsets of myeloid-biased cells [ 215 , 216 ]. Numerous studies on aging HSCs have examined heterogeneous HSC compartments containing a variety of subtypes, making it difficult to draw clear conclusions about age-related changes in specific, highly enriched HSC subsets. Additionally, research on aging in mouse models often contrasts young adult mice (2–4 months old) with older mice (1.5-2 years old). It’s important to note that HSCs transition from a fetal to an adult phenotype approximately one month after birth, during a phase characterized by rapid tissue growth and high self-renewal activity [ 224 , 225 ]. This developmental stage may contribute to the higher incidence of lymphoid malignancies observed in children, highlighting the need to include the juvenile period in studies of age-related alterations in HSCs [ 218 ]. Recent studies utilizing advanced single-cell sequencing techniques and more refined isolation methods have been initiated to address these challenges, offering deeper insights into the epigenetic and functional dynamics of HSCs across different life stages. This enhanced understanding is essential for identifying therapeutic targets to counteract age-related hematopoietic disorders [ 219 ].
HSC quiescence and proliferation with age
HSCs are getting more quiescent and undergo minimal proliferation as the age increases [ 219 ]. However, the cell cycle pertinent to these aged HSCs is yet unexplored vividly and debated [ 31 , 221 , 226 ], but as per the conclusions given by Tsu-Yi Su et al. juvenile mice typically undergo symmetric self-renewal process whereas aged HSCs could induce the generation of progenitors via symmetric proliferation [ 224 ]. Hence, in the aged HSCs, the enhanced production of progenitors aligns with stemness loss and mitigated functionality of HSCs [ 31 , 215 , 216 , 217 ]. Despite the reduction in this functionality, the phenotypic HSC population is enhanced in older age and maintains a larger population size by constraining the cell cycle process. Typically, the CD49b + HSCs were observed to be less quiescent and exhibits higher proliferation rate subsequently associated with minimal engraftment efficacy concluding to explore the CD49b– and CD49b + HSCs ability undergo symmetric or asymmetric division as the age increases; this exploration presenting a significant aspect for future research to uncover HSC heterogeneity as the age of the individuals increases.
Epigenetic regulation of HSC lineage bias
Lineage bias is considered to be a heritable trait [ 227 ] suggesting epigenetics may control heterogeneity of HSCs [ 228 ]. According to past reports, the functionally distinct HSCs do have identical gene expression patterns but display different epigenetic profiles. Single-cell sequencing/ATAC sequencing described the age-induced gene expression alteration and elucidated that juvenile HSCs have dissimilar molecular identity from fetal HSCs but are transcriptionally identical to adult HSCs. For instance, the chromatic accessibility of HSCs has eventually; the chromatin accessibility typically increased in both HSC subsets, correlating with the loss of SPIB and SPI1 (PU.1) transcription factor binding sites, consistent with the published reduction in PU.1 expression with age [ 220 , 222 , 223 , 229 ]. Mitigated SPIB TFBS is vividly observed in adult HSCs and suggests that certain gene expression alterations during aging originate from the juvenile stage typically at the time of the growth restriction process into adulthood [ 230 ]. Therefore, these kinds of age-mediated alterations could benefit to identification of epigenetics-associated signatures in age-related hematological malignancies. Subsequently, future studies are required to explore whether targeting chromatin remodeling across these epigenetic regions can reverse or mitigate aging-induced modifications in HSCs.
Subset-specific epigenetic differences
Predominantly, PU.1 levels are critical for myeloid bias; however, PU.1 deficiency in vivo models has been shown to enhance myelopoiesis while blocking lymphopoiesis, thereby increasing the propensity for myeloid leukemia as age increases [ 231 , 232 , 233 ]. These findings indicate that aging and lineage-biased differentiation may involve shared transcription factors. Furthermore, several other candidate genes have been identified in controlling HSC lineage bias. Specifically, Bcr, Abl1, and Tet1 are typically involved in both myeloid and lymphoid differentiation. In contrast, the role of Kcnn1 in the hematopoietic system remains unexplored. Modulations in the expression or mitigated expression of Bcr, Abl1, and Tet1 have been linked to hematopoietic malignancies, describing a crucial understanding of regulatory functions to modulate normal blood cell lineage differentiation [ 234 , 235 , 236 , 237 , 238 ]. Additional reports are necessary to elucidate the roles of these genes in regulating HSC lineage bias [ 219 ].
HSC aging research: Comprehensive genomic analysis of aging HSCs
To explore intrinsic aging mechanisms that compromise somatic stem cell function, we [ 220 ] conducted a detailed genomic analysis including histone analysis, and transcriptome/DNA analysis comparing young and aged murine HSCs. According to this analysis, a decrease in TGF-β signaling and disruptions in genes is critical for HSC proliferation or differentiation. Furthermore, aged HSCs displayed broader H3K4me3 peaks across genes associated with HSC self-renewal, alongside enhanced methylation rate of DNA typically at transcription factor binding sites of differentiation-promoting genes and decreased methylation at genes essential for HSC maintenance. These epigenetic modifications collectively reinforce self-renewal while diminishing differentiation, mirroring phenotypic aging in HSCs [ 220 ]. Additionally, ribosomal biogenesis is considered to be targeted during aging for modulating the ribosomal protein or RNA genes [ 220 ]. Previous reports offer a valuable resource for future epigenomic studies on stem cell aging. The observed epigenetic changes align with documented functional and phenotypic alterations in HSCs, such as increased self-renewal, reduced differentiation potential, and a myeloid-biased differentiation ratio. These modifications, though not directly pathological, create a cellular environment prone to age-related diseases like myelodysplastic syndrome (MDS) and leukemia [ 220 ].
Reduced TGF-β signaling in aged HSCs
A previous report described a significant reduction in TGF-β signaling pathways in aged HSCs, corroborating previous reports on aging in cardiac and neural tissues [ 239 , 240 ]. Differential expression of genes involved in the actuation of ligand and bioavailability include MMP-2 and MMP-9 [ 241 , 242 ], as well as mitigated levels of Smad6 expression, a gene interfering with Smad2 phosphorylation. These insights describe the necessity for further investigation into TGF-β pathway role in HSC aging.
Ribosomal gene transcription alterations
A report by Deqiang Su et al. [ 220 ] revealed significant alterations in ribosomal gene transcription with aging. Despite aged HSCs not being more proliferative than their younger counterparts [ 35 , 36 ], the increase in ribosomal protein gene transcription suggests enhanced splicing and potential translation efficiency. This finding aligns with studies linking ribosome biogenesis defects to bone marrow failure syndromes and malignancies like Diamond-Blackfan anemia [ 243 ]. Given the repeated association of ribosomal biogenesis with aging in various models [ 244 , 245 , 246 , 247 ], revisiting its role in mammalian stem cell aging is imperative.
H3K4me3 modifications and aging
Increased breadth of H3K4me3 peaks in aged HSCs, particularly on genes associated with self-renewal, suggests a correlation with functional declines observed in aging HSCs. This phenomenon mirrors observations in C. elegans, where mutations in H3K4 methylation genes extend longevity [ 248 ]. H3K4me3 accumulation on genes critical for self-renewal may drive the observed functional impairments in aged HSCs [ 249 , 250 ].
Linking HSC aging to myeloid malignancies
MDS and AML prevalence increases as the age increases, which is characterized by mitigated differentiation and a myeloid-lineage bias. Aging HSCs acquire changes predisposing them to these malignancies even without mutations. For instance, genes like Dnmt3a and Ezh2, often mutated in MDS and AML, show slight but significant downregulation with age [ 251 , 252 ]. Furthermore, hypomethylation and upregulation of HSC-specific genes (e.g., Gata2, Hmga2) and hypermethylation of differentiation-related transcription factors (e.g., Pu.1) suggest a predisposition towards malignant transformation. These observations warrant further investigation into the causal relationships between epigenetic changes and hematologic malignancies [ 251 , 252 , 253 , 254 ]. Developing novel interventions to modulate HSC function based on these insights holds promise for mitigating age-associated hematopoietic dysfunctions and improving health outcomes. By integrating these detailed genomic analyses and identifying specific future research directions, this discussion offers a comprehensive perspective on the mechanisms driving HSC aging and potential therapeutic strategies [ 220 ].
Aging, Diabetes, and obesity-induced BM alterations: hematopoiesis
Transcriptional dysregulation could be one of the factors that can promote aging. Cell cycle regulators [ 221 ], upregulated myeloid signatures [ 221 , 255 ], leukemia-causing genes [ 35 ], upregulation of inflammatory signaling through NF-kB, TNF-α [ 256 , 257 ], and mitigation in the DNA repair pathways [ 36 ] are predominantly accompanied by aging. Furthermore, the epigenetic alterations concomitant with transcriptional lesions are significantly observed in HSPCs derived from elderly individuals [ 220 , 258 ]. NTN1 I is a linchpin molecule actively involved in regulating BMEC-LepR + MSC niche A study by Pradeep Ramalingam et al. 2023 described the regulatory role of NTN1 in BMEC-LepR + MSC niche interactions inside the sinusoids of BM and ameliorate the accumulation of adipocytes [ 9 ]. Future studies are warranted to explore the role of BM niche-derived signaling to modulate the role of HSCs in vascular integrity, oxygenation, and adiposity inside the aging BM with hematological malignancies.
Leukemia: Insights into epigenetic regulation and clonal hematopoiesis in aging
Epigenetic regulation plays a crucial role in fostering transcriptional functions in stem cells in aging. Numerous clinical studies have identified gene mutations that could encode epigenetic enzymes in elderly individuals with oligoclonal hematopoiesis or patients with MDS or AML. The significant genes mainly affected are DNMT3A, EZH2, TET2, and SETDB1 [ 259 , 260 , 261 ]. Although the enzymatic activities of these proteins (DNA methylation/demethylation, H3K27/H3K9 tri-methylation) are well characterized, their specific effects on stem cell function remain unclear. It is elucidated that gene mutations pertinent to DNMT3A, EZH2, TET2, and SETDB1 can induce changes in the epigenetic memory of stem cells; this leads to modulation in transcriptional alterations which in turn fosters the self-renewal ability of mutant cells. Over time, these mutations promote clonal expansion within the bone marrow. Murine model-based reports described that changes in the expression of wild-type or mutate epigenetic writes can modulate the self-renewal process of HSCs [ 259 , 260 , 261 , 262 ].
Clonal hematopoiesis is a common phenomenon in older individuals. Initial studies, which examined X-chromosome inactivation patterns in the blood cells of elderly females, suggested that blood cells in older individuals originate from fewer stem cells [ 263 , 264 , 265 ]. More recent large-scale sequencing studies have confirmed these early observations and revealed that clonal hematopoiesis in healthy individuals increases the risk of developing leukemia, thereby associating it with increased mortality [ 265 ]. This raises critical questions about the detrimental nature of clonal hematopoiesis and its underlying mechanisms.
Despite its association with disease, clonal hematopoiesis is present in many healthy elderly individuals without any clinical symptoms [ 266 , 267 , 268 ]. This suggests that while clonal hematopoiesis can be linked to disease, it may also represent a benign aging process. Mutations in epigenetic genes might be a factor to drive clonal hematopoiesis might not be directly oncogenic but rather increase HSC self-renewal, leading to clonal expansion. When leukemic transformations occur, these actively self-renewing stem cells are more likely to acquire additional mutations, contributing to disease progression. Therefore, it is important to differentiate between mutations that drive benign clonal expansion and those that initiate leukemia.
Despite the established prevalence of clonal hematopoiesis in the elderly, the reports related to clonal stem cell impact on hematopoiesis are limited. A few reports proposed, to describe ranging from clonal succession to stochastic behavior, but no consensus has been reached [ 269 , 270 ]. This uncertainty arises because clonal descent of blood cells is historically difficult to trace. Early methods involved ex vivo barcoding of purified HSCs, followed by transplantation into recipient mice [ 271 , 272 , 273 ]. More recent approaches have used in vivo DNA barcoding and fluorescent dyes for clonal marking in transgenic mice and fish [ 274 , 275 ]. Exploring the roles of candidate genes identified in our ATAC-seq analysis, such as Bcr, Abl1, and Tet1, in regulating HSC lineage bias will be crucial for understanding the regulatory mechanisms pertinent to normal blood lineage differentiation and developing strategies to address hematopoietic malignancies associated with aging.
Understanding the functional impact of epigenetic changes on mature blood cells derived from aged stem cells will provide deeper insights into the links between clonal hematopoiesis and non-hematological diseases. Moreover, reconciling the differences in clonal contribution models and determining the precise number of stem cells contributing to steady-state hematopoiesis at the time of aging will be essential. Enhanced methods for tracing clonal descent in human studies, combined with experimental data from transgenic models, will be vital in addressing these gaps. Finally, translating these findings into clinical strategies to monitor and potentially modulate clonal hematopoiesis could lead to improved management of age-related hematopoietic and cardiovascular diseases [ 217 ].
Induces the reduction in the circulating BM-derived stem/progenitor cells and causes severe BM-related complications [ 10 , 276 , 277 ]. Inflammation has a significant role in the derangement of the BM microenvironment in the mouse models of type 1 diabetes as indicated by the presence of proinflammatory cytokines, and other monocyte/macrophage stimulating factors [ 278 ]. Changes in the cytokine expression in the long-term T1D conditions are accompanied by higher levels of IGF-1, IGFBP5, and osteoprotegerin and VEGF; concomitantly mitigated gene expression of Bmi1 could induce a decline in the HSPC senescence and fosters impairment of regenerative ability of HSC niche in the sinusoids of BM [ 10 , 279 ].
Disautonomia is associated with diabetes which could affect bone marrow function and damage to the peripheral clock. These events cause damage to the HSC niche during G-CSF-induced HSC mobilization in murine models [ 280 , 281 ]. Cardiovascular diabetic autonomic neuropathy is an abnormality that can induce mitigated levels of HSPCs with the upregulated expression of src homology & collagen homology domain (p66Shc), which consequently downregulates the expression of sirtuin 1 (Sirt1) [ 282 ]. Several in vivo studies delineated the diabetes-induced defects inside the microenvironment of BM including microangiography and neuropathy subsequently impair the stem cell mobilization egress. However, these mechanisms yet should be explored during hematological malignancies comorbid with diabetes.
Comorbid conditions such as obesity, and diabetes could foster proinflammatory conditions which could can induce CHIP-associated pathologies. The relationship between obesity and CHIP was described by Santosh P et al. [ 12 ]. In this study, authors elucidated the Ca2+/calcineurin-mediated Nfat and IRG1 signaling in the ob/ob fatty bone marrow recipient mice transplanted with Tet2–/– HSC/Ps which was accompanied by the substantial levels of itaconate [ 283 ], a metabolite involved in retrieving TCA cycle and impairs succinate dehydrogenase [ 284 ]. In addition, the obesity-mediated alterations in glucose availability induce a higher acetyl-CoA which concomitantly enhances the influx of divalent calcium into the cell. Subsequently, these events could cause activation and translocation of NFAT into the nucleus and result in the activation of lysine acetyltransferase p300 to foster the site-specific regulation of H3K27ac and promote Irg1 upregulated expression and cause accumulation of itaconate. This metabolite bonds to the alpha-ketoglutarate of the TET enzyme and impairs its catalytic activity at the time of DNA demethylation [ 285 , 286 ]. Santosh P et al. concluded that the transgenic Tet2-/-ob/ob mutant mice and chimeric ob/ob FBM recipient mice transplanted with Tet-/- HSC/Ps induced higher divalent calcium levels [ 12 ] and consequently declined the 5-hmC levels in their HSC/Ps; this a vivid role of obesity mediated CHIP-associated hematological malignancies are evident and the novel therapeutic modalities like gene-corrected stem cells may deliver effective clinical outcomes in the aging-associated mutated CHIP populations of BM and future studies are warranted to explore these modalities in clinical sectors.
Impact of atherosclerosis and myocardial infarction (MI) on the HSC niche
Haematopoiesis, the process by which mature immune cells are produced, plays a crucial role in responding to vascular injury, including in atherosclerosis and MI. During these cardiovascular events, infiltrating monocytes release pro-inflammatory cytokines such as TNFα, IL-1β, IL-6 and reactive oxygen species, which amplify local inflammation and promote further leukocyte recruitment. These monocytes also secrete metallopeptidases that contribute to pathogenic remodeling, such as destabilizing the fibrous cap in atherosclerosis and forming infarct scars in MI [ 287 , 288 ]. In the context of MI, monocyte infiltration occurs in two phases: an initial phase dominated by classical monocytes (Ly6hi CCR2hi in mice; CD14hi CD16 − in humans) that drive inflammation and phagocytosis, followed by a phase where nonclassical monocytes (Ly6Clo CX3CR1hi in mice; CD14lo CD16hi in humans) predominate to facilitate inflammation amelioration and cardiac remodeling. Nonclassical monocytes in this second phase are primarily derived from the conversion of classical monocytes, with minimal recruitment from the periphery [ 289 , 290 , 291 , 292 ].
Neutrophils, the most abundant circulating leukocytes, play a significant role in atherogenesis by releasing granules containing pro-inflammatory mediators, proteases, and enzymes that produce reactive oxygen species. These actions promote further leukocyte recruitment and the formation of neutrophil extracellular traps, which contribute to thrombosis. During MI, neutrophils also aid in angiogenesis and macrophage polarization toward an anti-inflammatory state, promoting cardiac tissue healing [ 290 ].
Correlation of MI and Clonal Hematopoiesis of Indeterminate Potential (CHIP)
As per the previous reports, the influence of post-MI heart failure on the vasculature of bone marrow elucidated that a significant reduction in type H bone endothelial cells (ECs), typically associated with inflammation due to the release of a higher IL-1β in type H vessels preceding their loss after MI. Blocking generation of IL-1β protected bone vascular niche, suggesting inflammatory signaling as the primary cause of type H vasculature reduction post-MI. It is speculated that IL-1β activation in CD31hiEMCNhi ECs can confer to pyroptosis and subsequently damage the endothelium of H vessels subsequently leading to age-induced damage to bone endothelium which further results in damage to hematopoiesis and activity of HSCs [ 151 , 293 , 294 , 295 ].
Given that MI could foster myeloid skewing and increase the number of LT-HSCs, it is plausible that MI could cause speedy HSC expansion with CHIP-driver mutations. In reality, individuals with ischemic heart disease are associated with a greater chance of incidence of CHIP-driver mutations when compared to age-matched cohorts [ 296 , 297 ]. An exploratory analysis from the CANTOS trial revealed that patients with CHIP mutations (e.g., TET2) experienced lower mortality than those without CHIP mutations [ 298 ].
To further elucidate the mechanisms underlying the interaction between cardiovascular diseases and the HSC niche, a detailed investigation into how cytokines and DAMPs interact with the NLRP3 inflammasome and other inflammatory pathways will be essential in understanding the progression from cardiovascular injury to hematopoietic dysregulation. Research should focus on how specific HSC subsets contribute to hematopoiesis during cardiovascular disease and aging. Investigating the differential adhesion and migration properties of these subsets could reveal targets for therapeutic intervention. On the other hand, conducting longitudinal studies using advanced clonal tracking techniques will help determine the stability and contribution of various HSC clones over time, particularly in the context of cardiovascular diseases. Expanding clinical trials to test the efficacy of anti-inflammatory treatments, like canakinumab, in broader populations and different stages of cardiovascular disease could provide new insights into managing CHIP and related hematopoietic anomalies. Furthermore, comprehensive profiling of genetic and epigenetic changes in HSCs from patients with cardiovascular diseases will help identify biomarkers for early detection and potential targets for personalized therapies. By addressing these areas, we can better understand and potentially mitigate the adverse effects of cardiovascular diseases on the hematopoietic system, improving outcomes for affected individuals [ 299 ].
Bi-directional impact of clonal hematopoiesis on the aging bone marrow niche
Understanding the bi-directional influences of CHIP on the aging bone marrow niche is crucial, as this interaction may elucidate the early changes that precede myeloid disease manifestation and potentially reveal targets for early intervention.
Inflammaging and Niche remodeling
A key concept in CHIP-related research is the acceleration of inflammaging, a chronic, low-grade inflammation associated with aging, which exacerbates BM niche remodeling beyond what is observed in normal aging. Genetic subtypes of CHIP, such as DNMT3A, TET2, and JAK2 mutations [ 300 ], have been linked to enhanced inflammatory responses. CHIP carriers exhibit typically enhanced proinflammatory cytokines, such as IL-6, IL-8, TNF, IL-1β, and IL-18 [ 268 , 301 , 302 ]. Gene-specific analyses highlight that TET2 mutations are associated with enhanced IL-1β, while JAK2 as well as SF3B1 mutations correlate with higher IL-18 levels [ 268 , 301 , 302 ]. This elucidates the necessity for gene-specific studies to understand the distinct inflammatory profiles induced by different CHIP mutations.
CHIP mutations also appear to influence bone structure, particularly in DNMT3A-mutant carriers, who are prone to osteoporosis. Proinflammatory cytokines from DNMT3A-deficient macrophages, such as IL-20, enhance osteoclastogenesis, leading to increased bone resorption and reduced bone mass, thereby deteriorating the endosteal niche [ 268 , 301 , 302 ]. Similarly, Tet2 inactivation in mice results in decreased bone mass, although the effects are less severe [ 268 , 301 , 302 ]. The involvement of growth factors like TGF-β and BMPs in bone resorption and MDS progression further illustrates the complex interplay between CHIP and bone health [ 303 , 304 ]. Emerging evidence suggests that CHIP mutations may impact MSC function and differentiation capacity. For instance, DNMT3A-mutant HSPCs induce MSC senescence via IL-6 production, which may result in clonal expansion and myeloid skewing [ 305 , 306 , 307 ]. Another condition including sympathetic neuropathy induced through mutant cells could also impair MSCs’ HSC-supportive function, highlighting a potential mechanism for CHIP-induced niche remodeling [ 305 , 306 , 307 ]. CHIP mutations may also promote vascular niche remodeling. Tet2-deficient immune cells, particularly myeloid cells, have been shown to enhance angiogenesis through increased S100A8/A9 secretion in a lung cancer model [ 308 ]. This pro-angiogenic and inflammatory environment likely increases BM vascularization and perivascular permeability, activating transcriptional activities pertinent to inflammation inside stromal cells as well as endothelial cells in BM during aging [ 267 , 309 ].
Selective advantage and clonal expansion
Aging and adipocyte-enriched BM is characterized by prolonged TNF signaling, which confers a selective advantage to DNMT3A- and TET2-mutant clones, facilitating their expansion [ 12 , 310 ]. In this case, IL-1R pathway is reported in driving Tet2+/− CHIP progression with aging [ 311 , 312 ]. Additionally, TET2 mutations may impair natural killer (NK) cell-mediated immune surveillance, further promoting the persistence of malignant clones [ 313 ].
The interplay between CHIP and the aging BM niche involves a continuous bi-directional inflammatory loop. Mutant HSPCs not only adapt to but also drive the remodeling of both the endosteal and vascular niches, creating a microenvironment that favors clonal expansion. This intricate relationship between intrinsic HSPC mutations and extrinsic niche factors elucidates the complexity of CHIP and its progression to hematologic malignancies [ 314 ]. Further studies should explore the molecular pathways through which CHIP mutations induce niche remodeling and inflammaging for identifying potential therapeutic targets to modulate the BM niche and inhibit CHIP progression. Developing strategies for early intervention in CHIP carriers is essential to prevent the transition to overt myeloid malignancies. In addition, it is advantageous to conduct gene-specific analyses to better understand the distinct inflammatory and remodeling responses induced by different CHIP mutations. By elucidating these mechanisms, we can develop targeted therapies to mitigate the adverse effects of CHIP on the aging BM niche and improve outcomes for individuals at risk of hematologic diseases [ 314 ].
Conclusions and future acknowledgments
Aging, obesity, and diabetes can cause significant alterations in the bone marrow. The factors concomitantly generated by these conditions can influence signaling across the BM, subsequently causing changes in hematopoiesis. These alterations can disrupt the normal function and regulation of HSCs and HSPCs, leading to an increased risk of hematological disorders and malignancies. Future studies are warranted to explore the following areas: Influence of autonomic signaling: Investigate how autonomic signaling affects the regulation of hematopoiesis, particularly in the context of aging, obesity, and diabetes. Understanding these mechanisms could reveal novel therapeutic targets to maintain healthy hematopoiesis. Age-related changes in hematopoiesis: Delve deeper into how aging-induced epigenetic influences hematopoietic processes at the cellular and molecular levels. Identifying specific age-related changes could help in developing strategies to counteract the adverse effects of aging on the hematopoietic system. Obesity-related clonal hematopoiesis: Study the impact of obesity on clonal hematopoiesis and its role in promoting hematological malignancies. Research in this area could lead to interventions that mitigate the risk of cancer in obese individuals by targeting the pathways involved in clonal expansion and mutation accumulation. Addressing these areas in future research could significantly advance our understanding of the complex interplay between systemic conditions like aging, obesity, diabetes, and hematopoietic regulation. This knowledge would be instrumental in developing novel therapeutic strategies to prevent and treat hematological malignancies associated with these conditions.
Abbreviations
Bone marrow
Tet methylcytosine dioxygenase 2
DNA (cytosine-5)-methyltransferase 3 A
ASXL transcriptional regulator 1
Janus kinase 2
C-X-C motif chemokine ligand 12
Stem cell factor
Bone marrow fibroblastoid colony-forming cells
Vascular endothelial growth factor
hematopoietic stem and progenitor cells
forkhead box P3
Intercellular adhesion molecule-1
B-cell-specific transcription repressor
early B cell factor-1
Paired Box (PAX) transcription factor 5
severe combined immunodeficiency
tumor necrosis factor
Runt-related transcription factor-2
Macrophage colony-stimulating factor
Multipotent long-term HSCs
Cell Division Cycle 42
Slit guidance ligand 2
insulin-like growth factor-1
Specificity protein-1
chemokine receptor 4
Beta-3-adrenergic receptors
Suppressor of Mothers against Decapentaplegic
Granulocyte-macrophage colony-stimulating factor
macrophage inhibitory protein-1 alpha
Sympathetic nervous system
Dipeptidyl peptidase 4
metalloproteinase
Bone Marrow endothelial cells
Insulin-like growth factor binding protein
Acute myeloid Leukaemia
Pittenger MF, Discher DE, Péault BM, Phinney DG, Hare JM, Caplan AI. <ArticleTitle Language=“En”>Mesenchymal stem cell perspective: cell biology to clinical progress. NPJ Regenerative Med. 2019;4(1):22.
Article Google Scholar
Wang L, Zhang H, Wang S, Chen X, Su J. Bone marrow adipocytes: a critical player in the bone marrow microenvironment. Front Cell Dev Biology. 2021;9:770705.
Kunisaki Y, Bruns I, Scheiermann C, Ahmed J, Pinho S, Zhang D, Mizoguchi T, Wei Q, Lucas D, Ito K. Arteriolar niches maintain haematopoietic stem cell quiescence. Nature. 2013;502(7473):637–43.
Article CAS PubMed PubMed Central Google Scholar
Ho Y-H, Del Toro R, Rivera-Torres J, Rak J, Korn C, García-García A, Macías D, González-Gómez C, Del Monte A, Wittner M. Remodeling of bone marrow hematopoietic stem cell niches promotes myeloid cell expansion during premature or physiological aging. Cell Stem Cell. 2019;25(3):407–18. e406.
Omatsu Y, Sugiyama T, Kohara H, Kondoh G, Fujii N, Kohno K, Nagasawa T. The essential functions of adipo-osteogenic progenitors as the hematopoietic stem and progenitor cell niche. Immunity. 2010;33(3):387–99.
Article CAS PubMed Google Scholar
Méndez-Ferrer S. Molecular interactome between HSCs and their niches. Blood. J Am Soc Hematol. 2019;134(15):1197–8.
Google Scholar
Mende N, Jolly A, Percin GI, Günther M, Rostovskaya M, Krishnan SM, Oostendorp RA, Dahl A, Anastassiadis K, Höfer T. Prospective isolation of nonhematopoietic cells of the niche and their differential molecular interactions with HSCs. Blood J Am Soc Hematol. 2019;134(15):1214–26.
Fielding C, Méndez-Ferrer S. Neuronal regulation of bone marrow stem cell niches. F1000Research 2020, 9.
Ramalingam P, Gutkin MC, Poulos MG, Tillery T, Doughty C, Winiarski A, Freire AG, Rafii S, Redmond D, Butler JM. Restoring bone marrow niche function rejuvenates aged hematopoietic stem cells by reactivating the DNA Damage Response. Nat Commun. 2023;14(1):2018.
Fadini GP, Ferraro F, Quaini F, Asahara T, Madeddu P. Concise review: diabetes, the bone marrow niche, and impaired vascular regeneration. Stem cells translational Med. 2014;3(8):949–57.
Article CAS Google Scholar
Oikawa A, Siragusa M, Quaini F, Mangialardi G, Katare RG, Caporali A, van Buul JD, van Alphen FP, Graiani G, Spinetti G. Diabetes mellitus induces bone marrow microangiopathy. Arterioscler Thromb Vasc Biol. 2010;30(3):498–508.
Pasupuleti SK, Ramdas B, Burns SS, Palam LR, Kanumuri R, Kumar R, Pandhiri TR, Dave UP, Yellapu NK, Zhou X. Obesity-induced inflammation exacerbates clonal hematopoiesis. J Clin Investig 2023, 133(11).
Lo Celso C, Fleming HE, Wu JW, Zhao CX, Miake-Lye S, Fujisaki J, Côté D, Rowe DW, Lin CP, Scadden DT. Live-animal tracking of individual haematopoietic stem/progenitor cells in their niche. Nature. 2009;457(7225):92–6.
Sipkins DA, Wei X, Wu JW, Runnels JM, Côté D, Means TK, Luster AD, Scadden DT, Lin CP. In vivo imaging of specialized bone marrow endothelial microdomains for tumour engraftment. Nature. 2005;435(7044):969–73.
Ding L, Morrison SJ. Haematopoietic stem cells and early lymphoid progenitors occupy distinct bone marrow niches. Nature. 2013;495(7440):231–5.
Ding L, Saunders TL, Enikolopov G, Morrison SJ. Endothelial and perivascular cells maintain haematopoietic stem cells. Nature. 2012;481(7382):457–62.
Méndez-Ferrer S, Michurina TV, Ferraro F, Mazloom AR, MacArthur BD, Lira SA, Scadden DT, Ma’ayan A, Enikolopov GN, Frenette PS. Mesenchymal and haematopoietic stem cells form a unique bone marrow niche. Nature. 2010;466(7308):829–34.
Article PubMed PubMed Central Google Scholar
Zhou X, Von Der Mark K, Henry S, Norton W, Adams H, De Crombrugghe B. Chondrocytes transdifferentiate into osteoblasts in endochondral bone during development, postnatal growth and fracture healing in mice. PLoS Genet. 2014;10(12):e1004820.
Xie Y, Yin T, Wiegraebe W, He XC, Miller D, Stark D, Perko K, Alexander R, Schwartz J, Grindley JC. Detection of functional haematopoietic stem cell niche using real-time imaging. Nature. 2009;457(7225):97–101.
Hooper AT, Butler JM, Nolan DJ, Kranz A, Iida K, Kobayashi M, Kopp H-G, Shido K, Petit I, Yanger K. Engraftment and reconstitution of hematopoiesis is dependent on VEGFR2-mediated regeneration of sinusoidal endothelial cells. Cell Stem Cell. 2009;4(3):263–74.
Till JE, McCulloch EA. A direct measurement of the radiation sensitivity of normal mouse bone marrow cells. Radiat Res. 2011;175(2):145–9.
Cheng T, Rodrigues N, Shen H, Yang Y-g, Dombkowski D, Sykes M, Scadden DT. Hematopoietic stem cell quiescence maintained by p21cip1/waf1. Science. 2000;287(5459):1804–8.
Zhao M, Perry JM, Marshall H, Venkatraman A, Qian P, He XC, Ahamed J, Li L. Megakaryocytes maintain homeostatic quiescence and promote post-injury regeneration of hematopoietic stem cells. Nat Med. 2014;20(11):1321–6.
Ye M, Zhang H, Amabile G, Yang H, Staber PB, Zhang P, Levantini E, Alberich-Jordà M, Zhang J, Kawasaki A. C/EBPa controls acquisition and maintenance of adult haematopoietic stem cell quiescence. Nat Cell Biol. 2013;15(4):385–94.
Lee JY, Hong S-H. Hematopoietic stem cells and their roles in tissue regeneration. Int J stem cells. 2020;13(1):1–12.
Wilson A, Laurenti E, Oser G, van der Wath RC, Blanco-Bose W, Jaworski M, Offner S, Dunant C, Eshkind L, Bockamp E. Hematopoietic Stem Cells Reversibly Switch from Dormancy to Self-Renewal during Homeostasis and Repairvol 135, pg 1118, (2008). Cell 2009, 138(1):209–209.
Harrison DE. Normal production of erythrocytes by mouse marrow continuous for 73 months. Proceedings of the National Academy of Sciences 1973, 70(11):3184–3188.
Yui J, Chiu C-P, Lansdorp PM. Telomerase activity in candidate stem cells from fetal liver and adult bone marrow. Blood J Am Soc Hematol. 1998;91(9):3255–62.
CAS Google Scholar
Geiger H, Van Zant G. The aging of lympho-hematopoietic stem cells. Nat Immunol. 2002;3(4):329–33.
Marley D, Goldman G. Evidence for a continuous decline in haemopoietic cell function from birth: application to evaluating bone marrow failure in children. Br J Haematol. 1999;106(1):162–6.
Morrison SJ, Wandycz AM, Akashi K, Globerson A, Weissman IL. The aging of hematopoietic stem cells. Nat Med. 1996;2(9):1011–6.
Liang Y, Van Zant G, Szilvassy SJ. Effects of aging on the homing and engraftment of murine hematopoietic stem and progenitor cells. Blood. 2005;106(4):1479–87.
Sudo K, Ema H, Morita Y, Nakauchi H. Age-associated characteristics of murine hematopoietic stem cells. J Exp Med. 2000;192(9):1273–80.
Kim M, MOON HB, Spangrude GJ. Major age-related changes of mouse hematopoietic stem/progenitor cells. Ann N Y Acad Sci. 2003;996(1):195–208.
Article PubMed Google Scholar
Rossi DJ, Bryder D, Zahn JM, Ahlenius H, Sonu R, Wagers AJ, Weissman IL. Cell intrinsic alterations underlie hematopoietic stem cell aging. Proc Natl Acad Sci. 2005;102(26):9194–9.
Chambers SM, Shaw CA, Gatza C, Fisk CJ, Donehower LA, Goodell MA. Aging hematopoietic stem cells decline in function and exhibit epigenetic dysregulation. PLoS Biol. 2007;5(8):e201.
Nijnik A, Woodbine L, Marchetti C, Dawson S, Lambe T, Liu C, Rodrigues NP, Crockford TL, Cabuy E, Vindigni A. DNA repair is limiting for haematopoietic stem cells during ageing. Nature. 2007;447(7145):686–90.
Rossi DJ, Bryder D, Seita J, Nussenzweig A, Hoeijmakers J, Weissman IL. Deficiencies in DNA damage repair limit the function of haematopoietic stem cells with age. Nature. 2007;447(7145):725–9.
Muller-Sieburg CE, Cho RH, Karlsson L, Huang J-F, Sieburg HB. Myeloid-biased hematopoietic stem cells have extensive self-renewal capacity but generate diminished lymphoid progeny with impaired IL-7 responsiveness. Blood. 2004;103(11):4111–8.
Muller-Sieburg CE, Sieburg HB. Clonal diversity of the stem cell compartment. Curr Opin Hematol. 2006;13(4):243–8.
Dykstra B, Kent D, Bowie M, McCaffrey L, Hamilton M, Lyons K, Lee S-J, Brinkman R, Eaves C. Long-term propagation of distinct hematopoietic differentiation programs in vivo. Cell Stem Cell. 2007;1(2):218–29.
Graf T. Two lineages of hematopoietic stem cells identified by differences in lineage priming. In: Experimental Hematology: 2007: ELSEVIER SCIENCE INC 360 PARK AVE SOUTH, NEW YORK, NY 10010 – 1710 USA; 2007: 1–2.
Muller-Sieburg CE, Cho RH, Thoman M, Adkins B, Sieburg HB. Deterministic regulation of hematopoietic stem cell self-renewal and differentiation. Blood J Am Soc Hematol. 2002;100(4):1302–9.
Cho RH, Müller-Sieburg CE. High frequency of long-term culture-initiating cells retain in vivo repopulation and self-renewal capacity. Exp Hematol. 2000;28(9):1080–6.
Cho RH, Sieburg HB, Muller-Sieburg CE. A new mechanism for the aging of hematopoietic stem cells: aging changes the clonal composition of the stem cell compartment but not individual stem cells. Blood J Am Soc Hematol. 2008;111(12):5553–61.
Frenette PS, Pinho S, Lucas D, Scheiermann C. Mesenchymal stem cell: keystone of the hematopoietic stem cell niche and a stepping-stone for regenerative medicine. Annu Rev Immunol. 2013;31:285–316.
Sacchetti B, Funari A, Michienzi S, Di Cesare S, Piersanti S, Saggio I, Tagliafico E, Ferrari S, Robey PG, Riminucci M. Self-renewing osteoprogenitors in bone marrow sinusoids can organize a hematopoietic microenvironment. Cell. 2007;131(2):324–36.
Chan CK, Chen C-C, Luppen CA, Kim J-B, DeBoer AT, Wei K, Helms JA, Kuo CJ, Kraft DL, Weissman IL. Endochondral ossification is required for haematopoietic stem-cell niche formation. Nature. 2009;457(7228):490–4.
Pinho S, Frenette PS. Haematopoietic stem cell activity and interactions with the niche. Nat Rev Mol Cell Biol. 2019;20(5):303–20.
Notta F, Doulatov S, Laurenti E, Poeppl A, Jurisica I, Dick JE. Isolation of single human hematopoietic stem cells capable of long-term multilineage engraftment. Science. 2011;333(6039):218–21.
Sugiyama T, Kohara H, Noda M, Nagasawa T. Maintenance of the hematopoietic stem cell pool by CXCL12-CXCR4 chemokine signaling in bone marrow stromal cell niches. Immunity. 2006;25(6):977–88.
Morikawa S, Mabuchi Y, Kubota Y, Nagai Y, Niibe K, Hiratsu E, Suzuki S, Miyauchi-Hara C, Nagoshi N, Sunabori T. Prospective identification, isolation, and systemic transplantation of multipotent mesenchymal stem cells in murine bone marrow. J Exp Med. 2009;206(11):2483–96.
Pinho S, Lacombe J, Hanoun M, Mizoguchi T, Bruns I, Kunisaki Y, Frenette PS. PDGFRα and CD51 mark human nestin + sphere-forming mesenchymal stem cells capable of hematopoietic progenitor cell expansion. J Exp Med. 2013;210(7):1351–67.
Tran E, Chinnasamy D, Yu Z, Morgan RA, Lee C-CR, Restifo NP, Rosenberg SA. Immune targeting of fibroblast activation protein triggers recognition of multipotent bone marrow stromal cells and cachexia. J Exp Med. 2013;210(6):1125–35.
Roberts EW, Deonarine A, Jones JO, Denton AE, Feig C, Lyons SK, Espeli M, Kraman M, McKenna B, Wells RJ. Depletion of stromal cells expressing fibroblast activation protein-α from skeletal muscle and bone marrow results in cachexia and anemia. J Exp Med. 2013;210(6):1137–51.
Kiel MJ, Yilmaz ÖH, Iwashita T, Yilmaz OH, Terhorst C, Morrison SJ. SLAM family receptors distinguish hematopoietic stem and progenitor cells and reveal endothelial niches for stem cells. Cell. 2005;121(7):1109–21.
Yao L, Yokota T, Xia L, Kincade PW, McEver RP. Bone marrow dysfunction in mice lacking the cytokine receptor gp130 in endothelial cells. Blood. 2005;106(13):4093–101.
Butler JM, Nolan DJ, Vertes EL, Varnum-Finney B, Kobayashi H, Hooper AT, Seandel M, Shido K, White IA, Kobayashi M. Endothelial cells are essential for the self-renewal and repopulation of Notch-dependent hematopoietic stem cells. Cell Stem Cell. 2010;6(3):251–64.
Kobayashi H, Butler JM, O’donnell R, Kobayashi M, Ding B-S, Bonner B, Chiu VK, Nolan DJ, Shido K, Benjamin L. Angiocrine factors from Akt-activated endothelial cells balance self-renewal and differentiation of haematopoietic stem cells. Nat Cell Biol. 2010;12(11):1046–56.
Winkler IG, Barbier V, Nowlan B, Jacobsen RN, Forristal CE, Patton JT, Magnani JL, Lévesque J-P. Vascular niche E-selectin regulates hematopoietic stem cell dormancy, self renewal and chemoresistance. Nat Med. 2012;18(11):1651–7.
Pierini A, Nishikii H, Baker J, Kimura T, Kwon H-S, Pan Y, Chen Y, Alvarez M, Strober W, Velardi A. Foxp3 + regulatory T cells maintain the bone marrow microenvironment for B cell lymphopoiesis. Nat Commun. 2017;8(1):15068.
Kaiser FM, Janowska I, Menafra R, de Gier M, Korzhenevich J, Pico-Knijnenburg I, Khatri I, Schulz A, Kuijpers TW, Lankester AC. IL-7 receptor signaling drives human B-cell progenitor differentiation and expansion. Blood 2023.
Sakaguchi S, Yamaguchi T, Nomura T, Ono M. Regulatory T cells and immune tolerance. Cell. 2008;133(5):775–87.
Pronk CJ, Veiby OP, Bryder D, Jacobsen SEW. Tumor necrosis factor restricts hematopoietic stem cell activity in mice: involvement of two distinct receptors. J Exp Med. 2011;208(8):1563–70.
Mizutani T, Tsuji K, Ebihara Y, Taki S, Ohba Y, Taniguchi T, Honda K. Homeostatic erythropoiesis by the transcription factor IRF2 through attenuation of type I interferon signaling. Exp Hematol. 2008;36(3):255–64.
Manz RA, Thiel A, Radbruch A. Lifetime of plasma cells in the bone marrow. Nature. 1997;388(6638):133–4.
Dulude G, Brochu S, Fontaine P, Baron C, Gyger M, Roy D, Perreault C. Thymic and extrathymic differentiation and expansion of T lymphocytes following bone marrow transplantation in irradiated recipients. Exp Hematol. 1997;25(9):992–1004.
CAS PubMed Google Scholar
Bolotin E, Smogorzewska M, Smith S, Widmer M, Weinberg K. Enhancement of thymopoiesis after bone marrow transplant by in vivo interleukin-7. 1996.
Crittenden SL, Bernstein DS, Bachorik JL, Thompson BE, Gallegos M, Petcherski AG, Moulder G, Barstead R, Wickens M, Kimble J. A conserved RNA-binding protein controls germline stem cells in Caenorhabditis elegans. Nature. 2002;417(6889):660–3.
Weissman IL. Stem cells: units of development, units of regeneration, and units in evolution. cell 2000, 100(1):157–168.
Kiel MJ, Morrison SJ. Uncertainty in the niches that maintain haematopoietic stem cells. Nat Rev Immunol. 2008;8(4):290–301.
Wu JY, Scadden DT, Kronenberg HM. Role of the osteoblast lineage in the bone marrow hematopoietic niches. J Bone Miner Res. 2009;24(5):759.
Lord BI, Testa NG, Hendry JH. The relative spatial distributions of CFUs and CFUc in the normal mouse femur. Blood. 1975;46(1):65–72.
Gong JK. Endosteal marrow: a rich source of hematopoietic stem cells. Science. 1978;199(4336):1443–5.
Zhu J, Garrett R, Jung Y, Zhang Y, Kim N, Wang J, Joe GJ, Hexner E, Choi Y, Taichman RS. Osteoblasts support B-lymphocyte commitment and differentiation from hematopoietic stem cells. Blood. 2007;109(9):3706–12.
Visnjic D, Kalajzic I, Gronowicz G, Aguila H, Clark S, Lichtler A, Rowe D. Conditional ablation of the osteoblast lineage in Col2. 3∆tk transgenic mice. J Bone Miner Res. 2001;16(12):2222–31.
Wu JY, Purton LE, Rodda SJ, Chen M, Weinstein LS, McMahon AP, Scadden DT, Kronenberg HM. Osteoblastic regulation of B lymphopoiesis is mediated by Gsα-dependent signaling pathways. Proceedings of the National Academy of Sciences 2008, 105(44):16976–16981.
Nagasawa T, Hirota S, Tachibana K, Takakura N, Nishikawa S-i, Kitamura Y, Yoshida N, Kikutani H, Kishimoto T. Defects of B-cell lymphopoiesis and bone-marrow myelopoiesis in mice lacking the CXC chemokine PBSF/SDF-1. Nature. 1996;382(6592):635–8.
Nagasawa T, Kikutani H, Kishimoto T. Molecular cloning and structure of a pre-B-cell growth-stimulating factor. Proc Natl Acad Sci. 1994;91(6):2305–9.
Nie Y, Han Y-C, Zou Y-R. CXCR4 is required for the quiescence of primitive hematopoietic cells. J Exp Med. 2008;205(4):777–83.
Massberg S, Schaerli P, Knezevic-Maramica I, Köllnberger M, Tubo N, Moseman EA, Huff IV, Junt T, Wagers AJ, Mazo IB. Physiological recirculation of hematopoietic stem and progenitor cells through blood, lymph and extramedullary tissues. Cell. 2007;131(5):994.
Singh P, Hu P, Hoggatt J, Moh A, Pelus LM. Expansion of bone marrow neutrophils following G-CSF administration in mice results in osteolineage cell apoptosis and mobilization of hematopoietic stem and progenitor cells. Leukemia. 2012;26(11):2375–83.
Cain DW, Snowden PB, Sempowski GD, Kelsoe G. Inflammation triggers emergency granulopoiesis through a density-dependent feedback mechanism. PLoS ONE. 2011;6(5):e19957.
Lévesque J-P, Hendy J, Takamatsu Y, Simmons PJ, Bendall LJ. Disruption of the CXCR4/CXCL12 chemotactic interaction during hematopoietic stem cell mobilization induced by GCSF or cyclophosphamide. J Clin Investig. 2003;111(2):187–96.
Aiuti A, Webb I, Bleul C, Springer T, Gutierrez-Ramos J. The chemokine SDF-1 is a chemoattractant for human CD34 + hematopoietic progenitor cells and provides a new mechanism to explain the mobilization of CD34 + progenitors to peripheral blood. J Exp Med. 1997;185(1):111–20.
Bendall LJ, Bradstock KF. G-CSF: From granulopoietic stimulant to bone marrow stem cell mobilizing agent. Cytokine Growth Factor Rev. 2014;25(4):355–67.
Semerad CL, Christopher MJ, Liu F, Short B, Simmons PJ, Winkler I, Levesque J-P, Chappel J, Ross FP, Link DC. G-CSF potently inhibits osteoblast activity and CXCL12 mRNA expression in the bone marrow. Blood. 2005;106(9):3020–7.
Liu F, Poursine-Laurent J, Link DC. Expression of the G-CSF receptor on hematopoietic progenitor cells is not required for their mobilization by G-CSF. Blood. J Am Soc Hematol. 2000;95(10):3025–31.
Christopher MJ, Rao M, Liu F, Woloszynek JR, Link DC. Expression of the G-CSF receptor in monocytic cells is sufficient to mediate hematopoietic progenitor mobilization by G-CSF in mice. J Exp Med. 2011;208(2):251–60.
Jacobsen RN, Forristal CE, Raggatt LJ, Nowlan B, Barbier V, Kaur S, van Rooijen N, Winkler IG, Pettit AR, Levesque J-P. Mobilization with granulocyte colony-stimulating factor blocks medullar erythropoiesis by depleting F4/80 + VCAM1 + CD169 + ER-HR3 + Ly6G + erythroid island macrophages in the mouse. Exp Hematol. 2014;42(7):547–61. e544.
Winkler I, Pettit A, Raggatt L, Jacobsen R, Forristal C, Barbier V, Nowlan B, Cisterne A, Bendall L, Sims N. Hematopoietic stem cell mobilizing agents G-CSF, cyclophosphamide or AMD3100 have distinct mechanisms of action on bone marrow HSC niches and bone formation. Leukemia. 2012;26(7):1594–601.
Chow A, Lucas D, Hidalgo A, Méndez-Ferrer S, Hashimoto D, Scheiermann C, Battista M, Leboeuf M, Prophete C, Van Rooijen N. Bone marrow CD169 + macrophages promote the retention of hematopoietic stem and progenitor cells in the mesenchymal stem cell niche. J Exp Med. 2011;208(2):261–71.
Winkler IG, Sims NA, Pettit AR, Barbier V, Nowlan B, Helwani F, Poulton IJ, van Rooijen N, Alexander KA, Raggatt LJ. Bone marrow macrophages maintain hematopoietic stem cell (HSC) niches and their depletion mobilizes HSCs. Blood J Am Soc Hematol. 2010;116(23):4815–28.
Hashimoto D, Chow A, Greter M, Saenger Y, Kwan W-H, Leboeuf M, Ginhoux F, Ochando JC, Kunisaki Y, van Rooijen N. Pretransplant CSF-1 therapy expands recipient macrophages and ameliorates GVHD after allogeneic hematopoietic cell transplantation. J Exp Med. 2011;208(5):1069–82.
Li Z, Xu X, Feng X, Murphy PM. The macrophage-depleting agent clodronate promotes durable hematopoietic chimerism and donor-specific skin allograft tolerance in mice. Sci Rep. 2016;6(1):22143.
Kaur S, Raggatt LJ, Millard SM, Wu AC, Batoon L, Jacobsen RN, Winkler IG, MacDonald KP, Perkins AC, Hume DA. Self-repopulating recipient bone marrow resident macrophages promote long-term hematopoietic stem cell engraftment. Blood J Am Soc Hematol. 2018;132(7):735–49.
Lehenkari PP, Kellinsalmi M, Näpänkangas JP, Ylitalo KV, Mönkkönen J, Rogers MJ, Azhayev A, Väänänen HK, Hassinen IE. Further insight into mechanism of action of clodronate: inhibition of mitochondrial ADP/ATP translocase by a nonhydrolyzable, adenine-containing metabolite. Mol Pharmacol. 2002;61(5):1255–62.
Chow A, Huggins M, Ahmed J, Hashimoto D, Lucas D, Kunisaki Y, Pinho S, Leboeuf M, Noizat C, Van Rooijen N. CD169 + macrophages provide a niche promoting erythropoiesis under homeostasis and stress. Nat Med. 2013;19(4):429–36.
Da Bandeira DS, Casamitjana J, Crisan M. Pericytes, integral components of adult hematopoietic stem cell niches. Pharmacol Ther. 2017;171:104–13.
Long F, Ornitz DM. Development of the endochondral skeleton. Cold Spring Harb Perspect Biol. 2013;5(1):a008334.
Eshkar-Oren I, Viukov SV, Salameh S, Krief S, Oh C-d, Akiyama H, Gerber H-P, Ferrara N, Zelzer E. The forming limb skeleton serves as a signaling center for limb vasculature patterning via regulation of Vegf. 2009.
Maes C, Carmeliet P, Moermans K, Stockmans I, Smets N, Collen D, Bouillon R, Carmeliet G. Impaired angiogenesis and endochondral bone formation in mice lacking the vascular endothelial growth factor isoforms VEGF164 and VEGF188. Mech Dev. 2002;111(1–2):61–73.
Ding B-S, Cao Z, Lis R, Nolan DJ, Guo P, Simons M, Penfold ME, Shido K, Rabbany SY, Rafii S. Divergent angiocrine signals from vascular niche balance liver regeneration and fibrosis. Nature. 2014;505(7481):97–102.
Ding B-S, Nolan DJ, Butler JM, James D, Babazadeh AO, Rosenwaks Z, Mittal V, Kobayashi H, Shido K, Lyden D. Inductive angiocrine signals from sinusoidal endothelium are required for liver regeneration. Nature. 2010;468(7321):310–5.
Ding B-S, Nolan DJ, Guo P, Babazadeh AO, Cao Z, Rosenwaks Z, Crystal RG, Simons M, Sato TN, Worgall S. Endothelial-derived angiocrine signals induce and sustain regenerative lung alveolarization. Cell. 2011;147(3):539–53.
Ramasamy SK, Kusumbe AP, Wang L, Adams RH. Endothelial Notch activity promotes angiogenesis and osteogenesis in bone. Nature. 2014;507(7492):376–80.
Hu J, Srivastava K, Wieland M, Runge A, Mogler C, Besemfelder E, Terhardt D, Vogel MJ, Cao L, Korn C. Endothelial cell-derived angiopoietin-2 controls liver regeneration as a spatiotemporal rheostat. Science. 2014;343(6169):416–9.
Kusumbe AP, Ramasamy SK, Adams RH. Coupling of angiogenesis and osteogenesis by a specific vessel subtype in bone. Nature. 2014;507(7492):323–8.
Langen UH, Pitulescu ME, Kim JM, Enriquez-Gasca R, Sivaraj KK, Kusumbe AP, Singh A, Di Russo J, Bixel MG, Zhou B. Cell–matrix signals specify bone endothelial cells during developmental osteogenesis. Nat Cell Biol. 2017;19(3):189–201.
Frenette PS, Pinho S, Lucas D, Scheiermann C. Mesenchymal stem cell: keystone of the hematopoietic stem cell niche and a stepping-stone for regenerative medicine. Annu Rev Immunol. 2013;31(1):285–316.
Scheller EL, Cawthorn WP, Burr AA, Horowitz MC, MacDougald OA. Marrow adipose tissue: trimming the fat. Trends Endocrinol Metabolism. 2016;27(6):392–403.
Naveiras O, Nardi V, Wenzel PL, Hauschka PV, Fahey F, Daley GQ. Bone-marrow adipocytes as negative regulators of the haematopoietic microenvironment. Nature. 2009;460(7252):259–63.
Oguro H, Ding L, Morrison SJ. SLAM family markers resolve functionally distinct subpopulations of hematopoietic stem cells and multipotent progenitors. Cell Stem Cell. 2013;13(1):102–16.
Zhou BO, Yu H, Yue R, Zhao Z, Rios JJ, Naveiras O, Morrison SJ. Bone marrow adipocytes promote the regeneration of stem cells and haematopoiesis by secreting SCF. Nat Cell Biol. 2017;19(8):891–903.
DiMascio L, Voermans C, Uqoezwa M, Duncan A, Lu D, Wu J, Sankar U, Reya T. Identification of adiponectin as a novel hemopoietic stem cell growth factor. J Immunol. 2007;178(6):3511–20.
Poloni A, Maurizi G, Serrani F, Mancini S, Zingaretti MC, Frontini A, Cinti S, Olivieri A, Leoni P. Molecular and functional characterization of human bone marrow adipocytes. Exp Hematol. 2013;41(6):558–66. e552.
Robino JJ, Pamir N, Rosario S, Crawford LB, Burwitz BJ, Roberts CT Jr, Kurre P, Varlamov O. Spatial and biochemical interactions between bone marrow adipose tissue and hematopoietic stem and progenitor cells in rhesus macaques. Bone. 2020;133:115248.
Cahu X, Calvo J, Poglio S, Prade N, Colsch B, Arcangeli M-L, Leblanc T, Petit A, Baleydier F, Baruchel A. Bone marrow sites differently imprint dormancy and chemoresistance to T-cell acute lymphoblastic leukemia. Blood Adv. 2017;1(20):1760–72.
Shafat MS, Oellerich T, Mohr S, Robinson SD, Edwards DR, Marlein CR, Piddock RE, Fenech M, Zaitseva L, Abdul-Aziz A. Leukemic blasts program bone marrow adipocytes to generate a protumoral microenvironment. Blood J Am Soc Hematol. 2017;129(10):1320–32.
Boyd AL, Reid JC, Salci KR, Aslostovar L, Benoit YD, Shapovalova Z, Nakanishi M, Porras DP, Almakadi M, Campbell CJ. Acute myeloid leukaemia disrupts endogenous myelo-erythropoiesis by compromising the adipocyte bone marrow niche. Nat Cell Biol. 2017;19(11):1336–47.
Zinngrebe J, Debatin K-M, Fischer-Posovszky P. Adipocytes in hematopoiesis and acute leukemia: friends, enemies, or innocent bystanders? Leukemia. 2020;34(9):2305–16.
Otto F, Thornell AP, Crompton T, Denzel A, Gilmour KC, Rosewell IR, Stamp GW, Beddington RS, Mundlos S, Olsen BR. Cbfa1, a candidate gene for cleidocranial dysplasia syndrome, is essential for osteoblast differentiation and bone development. Cell. 1997;89(5):765–71.
Komori T, Yagi H, Nomura S, Yamaguchi A, Sasaki K, Deguchi K, Shimizu Y, Bronson R, Gao Y-H, Inada M. Targeted disruption of Cbfa1 results in a complete lack of bone formation owing to maturational arrest of osteoblasts. Cell. 1997;89(5):755–64.
Deguchi K, Yagi H, Inada M, Yoshizaki K, Kishimoto T, Komori T. Excessive extramedullary hematopoiesis in Cbfa1-deficient mice with a congenital lack of bone marrow. Biochem Biophys Res Commun. 1999;255(2):352–9.
Yoshida H, Hayashi S-I, Kunisada T, Ogawa M, Nishikawa S, Okamura H, Sudo T, Shultz LD, Nishikawa S-I. The murine mutation osteopetrosis is in the coding region of the macrophage colony stimulating factor gene. Nature. 1990;345(6274):442–4.
Sutherland HJ, Lansdorp PM, Henkelman DH, Eaves AC, Eaves CJ. Functional characterization of individual human hematopoietic stem cells cultured at limiting dilution on supportive marrow stromal layers. Proc Natl Acad Sci. 1990;87(9):3584–8.
Taichman RS, Reilly MJ, Emerson SG. Human osteoblasts support human hematopoietic progenitor cells in vitro bone marrow cultures. 1996.
Taichman RS, Emerson SG. The role of osteoblasts in the hematopoietic microenvironment. Stem Cells. 1998;16(1):7–15.
Visnjic D, Kalajzic Z, Rowe DW, Katavic V, Lorenzo J, Aguila HL. Hematopoiesis is severely altered in mice with an induced osteoblast deficiency. Blood. 2004;103(9):3258–64.
Katayama Y, Battista M, Kao W-M, Hidalgo A, Peired AJ, Thomas SA, Frenette PS. Signals from the sympathetic nervous system regulate hematopoietic stem cell egress from bone marrow. Cell. 2006;124(2):407–21.
Mayack SR, Wagers AJ. Osteolineage niche cells initiate hematopoietic stem cell mobilization. Blood J Am Soc Hematol. 2008;112(3):519–31.
Calvi L, Adams G, Weibrecht K, Weber J, Olson D, Knight M, Martin R, Schipani E, Divieti P, Bringhurst FR. Osteoblastic cells regulate the haematopoietic stem cell niche. Nature. 2003;425(6960):841–6.
Zhang J, Niu C, Ye L, Huang H, He X, Tong W-G, Ross J, Haug J, Johnson T, Feng JQ. Identification of the haematopoietic stem cell niche and control of the niche size. Nature. 2003;425(6960):836–41.
Kiel MJ, Radice GL, Morrison SJ. Lack of evidence that hematopoietic stem cells depend on N-cadherin-mediated adhesion to osteoblasts for their maintenance. Cell Stem Cell. 2007;1(2):204–17.
Arai F, Hirao A, Ohmura M, Sato H, Matsuoka S, Takubo K, Ito K, Koh GY, Suda T. Tie2/angiopoietin-1 signaling regulates hematopoietic stem cell quiescence in the bone marrow niche. Cell. 2004;118(2):149–61.
Stier S, Ko Y, Forkert R, Lutz C, Neuhaus T, Grünewald E, Cheng T, Dombkowski D, Calvi LM, Rittling SR. Osteopontin is a hematopoietic stem cell niche component that negatively regulates stem cell pool size. J Exp Med. 2005;201(11):1781–91.
Nilsson SK, Johnston HM, Whitty GA, Williams B, Webb RJ, Denhardt DT, Bertoncello I, Bendall LJ, Simmons PJ, Haylock DN. Osteopontin, a key component of the hematopoietic stem cell niche and regulator of primitive hematopoietic progenitor cells. Blood. 2005;106(4):1232–9.
Yoshihara H, Arai F, Hosokawa K, Hagiwara T, Takubo K, Nakamura Y, Gomei Y, Iwasaki H, Matsuoka S, Miyamoto K. Thrombopoietin/MPL signaling regulates hematopoietic stem cell quiescence and interaction with the osteoblastic niche. Cell Stem Cell. 2007;1(6):685–97.
Fleming HE, Janzen V, Celso CL, Guo J, Leahy KM, Kronenberg HM, Scadden DT. Wnt signaling in the niche enforces hematopoietic stem cell quiescence and is necessary to preserve self-renewal in vivo. Cell Stem Cell. 2008;2(3):274–83.
Adams GB, Chabner KT, Alley IR, Olson DP, Szczepiorkowski ZM, Poznansky MC, Kos CH, Pollak MR, Brown EM, Scadden DT. Stem cell engraftment at the endosteal niche is specified by the calcium-sensing receptor. Nature. 2006;439(7076):599–603.
Mancini SJ, Mantei N, Dumortier A, Suter U, MacDonald HR, Radtke F. Jagged1-dependent Notch signaling is dispensable for hematopoietic stem cell self-renewal and differentiation. Blood. 2005;105(6):2340–2.
Arai F, Suda T. Maintenance of quiescent hematopoietic stem cells in the osteoblastic niche. Ann N Y Acad Sci. 2007;1106(1):41–53.
Niu C, Zhang J, Breslin P, Onciu M, Ma Z, Morris SW. c-Myc is a target of RNA-binding motif protein 15 in the regulation of adult hematopoietic stem cell and megakaryocyte development. Blood. J Am Soc Hematol. 2009;114(10):2087–96.
Wilson A, Murphy MJ, Oskarsson T, Kaloulis K, Bettess MD, Oser GM, Pasche A-C, Knabenhans C, MacDonald HR. Trumpp A: c-Myc controls the balance between hematopoietic stem cell self-renewal and differentiation. Genes Dev. 2004;18(22):2747–63.
Wein F, Pietsch L, Saffrich R, Wuchter P, Walenda T, Bork S, Horn P, Diehlmann A, Eckstein V, Ho AD. N-cadherin is expressed on human hematopoietic progenitor cells and mediates interaction with human mesenchymal stromal cells. Stem cell Res. 2010;4(2):129–39.
Maryanovich M, Zahalka AH, Pierce H, Pinho S, Nakahara F, Asada N, Wei Q, Wang X, Ciero P, Xu J. Adrenergic nerve degeneration in bone marrow drives aging of the hematopoietic stem cell niche. Nat Med. 2018;24(6):782–91.
Kusumbe AP, Ramasamy SK, Itkin T, Mäe MA, Langen UH, Betsholtz C, Lapidot T, Adams RH. Age-dependent modulation of vascular niches for haematopoietic stem cells. Nature. 2016;532(7599):380–4.
Ambrosi TH, Scialdone A, Graja A, Gohlke S, Jank A-M, Bocian C, Woelk L, Fan H, Logan DW, Schürmann A. Adipocyte accumulation in the bone marrow during obesity and aging impairs stem cell-based hematopoietic and bone regeneration. Cell Stem Cell. 2017;20(6):771–84. e776.
Guidi N, Sacma M, Ständker L, Soller K, Marka G, Eiwen K, Weiss JM, Kirchhoff F, Weil T, Cancelas JA. Osteopontin attenuates aging-associated phenotypes of hematopoietic stem cells. EMBO J. 2017;36(7):840–53.
Ergen AV, Boles NC, Goodell MA. Rantes/Ccl5 influences hematopoietic stem cell subtypes and causes myeloid skewing. Blood J Am Soc Hematol. 2012;119(11):2500–9.
Poulos MG, Ramalingam P, Gutkin MC, Llanos P, Gilleran K, Rabbany SY, Butler JM. Endothelial transplantation rejuvenates aged hematopoietic stem cell function. J Clin Investig. 2017;127(11):4163–78.
Powell ND, Sloan EK, Bailey MT, Arevalo JM, Miller GE, Chen E, Kobor MS, Reader BF, Sheridan JF, Cole SW. Social stress up-regulates inflammatory gene expression in the leukocyte transcriptome via β-adrenergic induction of myelopoiesis. Proceedings of the National Academy of Sciences 2013, 110(41):16574–16579.
Heidt T, Sager HB, Courties G, Dutta P, Iwamoto Y, Zaltsman A, von Zur Muhlen C, Bode C, Fricchione GL, Denninger J. Chronic variable stress activates hematopoietic stem cells. Nat Med. 2014;20(7):754–8.
Jin J, Wang X, Wang Q, Guo X, Cao J, Zhang X, Zhu T, Zhang D, Wang W, Wang J. Chronic psychological stress induces the accumulation of myeloid-derived suppressor cells in mice. PLoS ONE. 2013;8(9):e74497.
Hasan S, Johnson NB, Mosier MJ, Shankar R, Conrad P, Szilagyi A, Gamelli RL, Muthumalaiappan K. Myelo-erythroid commitment after burn injury is under β-adrenergic control via MafB regulation. Am J Physiology-Cell Physiol. 2017;312(3):C286–301.
Himburg HA, Muramoto GG, Daher P, Meadows SK, Russell JL, Doan P, Chi J-T, Salter AB, Lento WE, Reya T. Pleiotrophin regulates the expansion and regeneration of hematopoietic stem cells. Nat Med. 2010;16(4):475–82.
Himburg HA, Harris JR, Ito T, Daher P, Russell JL, Quarmyne M, Doan PL, Helms K, Nakamura M, Fixsen E. Pleiotrophin regulates the retention and self-renewal of hematopoietic stem cells in the bone marrow vascular niche. Cell Rep. 2012;2(4):964–75.
Smith-Berdan S, Nguyen A, Hassanein D, Zimmer M, Ugarte F, Ciriza J, Li D, García-Ojeda ME, Hinck L, Forsberg EC. Robo4 cooperates with CXCR4 to specify hematopoietic stem cell localization to bone marrow niches. Cell Stem Cell. 2011;8(1):72–83.
Smith-Berdan S, Schepers K, Ly A, Passegué E, Forsberg EC. Dynamic expression of the Robo ligand Slit2 in bone marrow cell populations. Cell Cycle. 2012;11(4):675–82.
Nakamura-Ishizu A, Okuno Y, Omatsu Y, Okabe K, Morimoto J, Uede T, Nagasawa T, Suda T, Kubota Y. Extracellular matrix protein tenascin-C is required in the bone marrow microenvironment primed for hematopoietic regeneration. Blood J Am Soc Hematol. 2012;119(23):5429–37.
Taichman RS, Emerson SG. Human osteoblasts support hematopoiesis through the production of granulocyte colony-stimulating factor. J Exp Med. 1994;179(5):1677–82.
Kollet O, Dar A, Shivtiel S, Kalinkovich A, Lapid K, Sztainberg Y, Tesio M, Samstein RM, Goichberg P, Spiegel A. Osteoclasts degrade endosteal components and promote mobilization of hematopoietic progenitor cells. Nat Med. 2006;12(6):657–64.
Mangialardi G, Cordaro A, Madeddu P. The bone marrow pericyte: an orchestrator of vascular niche. Regen Med. 2016;11(8):883–95.
Li W, Johnson SA, Shelley WC, Yoder MC. Hematopoietic stem cell repopulating ability can be maintained in vitro by some primary endothelial cells. Exp Hematol. 2004;32(12):1226–37.
Sreeramkumar V, Leiva M, Stadtmann A, Pitaval C, Ortega-Rodríguez I, Wild MK, Lee B, Zarbock A, Hidalgo A. Coordinated and unique functions of the E-selectin ligand ESL-1 during inflammatory and hematopoietic recruitment in mice. Blood J Am Soc Hematol. 2013;122(24):3993–4001.
Yamazaki S, Ema H, Karlsson G, Yamaguchi T, Miyoshi H, Shioda S, Taketo MM, Karlsson S, Iwama A, Nakauchi H. Nonmyelinating Schwann cells maintain hematopoietic stem cell hibernation in the bone marrow niche. Cell. 2011;147(5):1146–58.
Karanu FN, Murdoch B, Gallacher L, Wu DM, Koremoto M, Sakano S, Bhatia M. The notch ligand jagged-1 represents a novel growth factor of human hematopoietic stem cells. J Exp Med. 2000;192(9):1365–72.
Nemeth MJ, Topol L, Anderson SM, Yang Y, Bodine DM. Wnt5a inhibits canonical Wnt signaling in hematopoietic stem cells and enhances repopulation. Proceedings of the National Academy of Sciences 2007, 104(39):15436–15441.
Zhang P, Zhang C, Li J, Han J, Liu X, Yang H. The physical microenvironment of hematopoietic stem cells and its emerging roles in engineering applications. Stem Cell Res Ther. 2019;10:1–13.
Beeraka NM, Basappa B, Nikolenko VN, Mahesh P. Role of Neurotransmitters in Steady State Hematopoiesis, Aging, and Leukemia. Stem Cell Reviews Rep 2024:1–26.
Chen Q, Shou P, Zheng C, Jiang M, Cao G, Yang Q, Cao J, Xie N, Velletri T, Zhang X. Fate decision of mesenchymal stem cells: adipocytes or osteoblasts? Cell Death Differ. 2016;23(7):1128–39.
Kang Q, Song W-X, Luo Q, Tang N, Luo J, Luo X, Chen J, Bi Y, He B-C, Park JK. A comprehensive analysis of the dual roles of BMPs in regulating adipogenic and osteogenic differentiation of mesenchymal progenitor cells. Stem Cells Dev. 2009;18(4):545–58.
Deng Z-L, Sharff KA, Tang N, Song W-X, Luo J, Luo X, Chen J, Bennett E, Reid R, Manning D. Regulation of osteogenic differentiation during skeletal development. Front Biosci. 2008;13(1):2001–21.
Chen G, Deng C, Li Y-P. TGF-β and BMP signaling in osteoblast differentiation and bone formation. Int J Biol Sci. 2012;8(2):272.
Yuan Z, Li Q, Luo S, Liu Z, Luo D, Zhang B, Zhang D, Rao P, Xiao J. PPARγ and Wnt signaling in adipogenic and osteogenic differentiation of mesenchymal stem cells. Curr Stem Cell Res Therapy. 2016;11(3):216–25.
Park HW, Kim YC, Yu B, Moroishi T, Mo J-S, Plouffe SW, Meng Z, Lin KC, Yu F-X, Alexander CM. Alternative Wnt signaling activates YAP/TAZ. Cell. 2015;162(4):780–94.
Byun M, Hwang J, Kim A, Kim K, Hwang E, Yaffe M, Hong J. Canonical Wnt signalling activates TAZ through PP1A during osteogenic differentiation. Cell Death Differ. 2014;21(6):854–63.
Bennett CN, Ouyang H, Ma YL, Zeng Q, Gerin I, Sousa KM, Lane TF, Krishnan V, Hankenson KD, MacDougald OA. Wnt10b increases postnatal bone formation by enhancing osteoblast differentiation. J Bone Miner Res. 2007;22(12):1924–32.
Stevens JR, Miranda-Carboni GA, Singer MA, Brugger SM, Lyons KM, Lane TF. Wnt10b deficiency results in age‐dependent loss of bone mass and progressive reduction of mesenchymal progenitor cells. J Bone Miner Res. 2010;25(10):2138–47.
Arango NA, Szotek PP, Manganaro TF, Oliva E, Donahoe PK, Teixeira J. Conditional deletion of β-catenin in the mesenchyme of the developing mouse uterus results in a switch to adipogenesis in the myometrium. Dev Biol. 2005;288(1):276–83.
Song B-q, Chi Y, Li X, Du W-j, Han Z-B, Tian J-j, Li J-j, Chen F, Wu H-h. Han L-x: Inhibition of Notch signaling promotes the adipogenic differentiation of mesenchymal stem cells through autophagy activation and PTEN-PI3K/AKT/mTOR pathway. Cell Physiol Biochem. 2015;36(5):1991–2002.
Shimizu T, Tanaka T, Iso T, Matsui H, Ooyama Y, Kawai-Kowase K, Arai M, Kurabayashi M. Notch signaling pathway enhances bone morphogenetic protein 2 (BMP2) responsiveness of Msx2 gene to induce osteogenic differentiation and mineralization of vascular smooth muscle cells. J Biol Chem. 2011;286(21):19138–48.
Fontaine C, Cousin W, Plaisant M, Dani C, Peraldi P. Hedgehog signaling alters adipocyte maturation of human mesenchymal stem cells. Stem Cells. 2008;26(4):1037–46.
Kim WK, Meliton V, Bourquard N, Hahn TJ, Parhami F. Hedgehog signaling and osteogenic differentiation in multipotent bone marrow stromal cells are inhibited by oxidative stress. J Cell Biochem. 2010;111(5):1199–209.
James AW, Pang S, Askarinam A, Corselli M, Zara JN, Goyal R, Chang L, Pan A, Shen J, Yuan W. Additive effects of sonic hedgehog and Nell-1 signaling in osteogenic versus adipogenic differentiation of human adipose-derived stromal cells. Stem Cells Dev. 2012;21(12):2170–8.
Li L, Dong Q, Wang Y, Feng Q, Zhou P, Ou X, Meng Q, He T, Luo J. Hedgehog signaling is involved in the BMP9-induced osteogenic differentiation of mesenchymal stem cells Retraction in/10.3892/ijmm. 2023.5233. International journal of molecular medicine 2015, 35(6):1641–1650.
Meunier P, Aaron J, Edouard C, VlGNON G. Osteoporosis and the replacement of cell populations of the marrow by adipose tissue: a quantitative study of 84 iliac bone biopsies. Clin Orthop Relat Research ® . 1971;80:147–54.
Justesen J, Stenderup K, Ebbesen E, Mosekilde L, Steiniche T, Kassem M. Adipocyte tissue volume in bone marrow is increased with aging and in patients with osteoporosis. Biogerontology. 2001;2:165–71.
Moerman EJ, Teng K, Lipschitz DA, Lecka-Czernik B. Aging activates adipogenic and suppresses osteogenic programs in mesenchymal marrow stroma/stem cells: the role of PPAR‐γ2 transcription factor and TGF‐β/BMP signaling pathways. Aging Cell. 2004;3(6):379–89.
Almeida M, Ambrogini E, Han L, Manolagas SC, Jilka RL. Increased lipid oxidation causes oxidative stress, increased peroxisome proliferator-activated receptor-γ expression, and diminished pro-osteogenic Wnt signaling in the skeleton. J Biol Chem. 2009;284(40):27438–48.
Kousteni S. FoxO1, the transcriptional chief of staff of energy metabolism. Bone. 2012;50(2):437–43.
Schaum N, Lehallier B, Hahn O, Pálovics R, Hosseinzadeh S, Lee SE, Sit R, Lee DP, Losada PM, Zardeneta ME. Ageing hallmarks exhibit organ-specific temporal signatures. Nature. 2020;583(7817):596–602.
Beerman I, Bhattacharya D, Zandi S, Sigvardsson M, Weissman IL, Bryder D, Rossi DJ. Functionally distinct hematopoietic stem cells modulate hematopoietic lineage potential during aging by a mechanism of clonal expansion. Proceedings of the National Academy of Sciences 2010, 107(12):5465–5470.
Säwén P, Lang S, Mandal P, Rossi DJ, Soneji S, Bryder D. Mitotic history reveals distinct stem cell populations and their contributions to hematopoiesis. Cell Rep. 2016;14(12):2809–18.
Ho TT, Warr MR, Adelman ER, Lansinger OM, Flach J, Verovskaya EV, Figueroa ME, Passegué E. Autophagy maintains the metabolism and function of young and old stem cells. Nature. 2017;543(7644):205–10.
Florian MC, Dörr K, Niebel A, Daria D, Schrezenmeier H, Rojewski M, Filippi M-D, Hasenberg A, Gunzer M, Scharffetter-Kochanek K. Cdc42 activity regulates hematopoietic stem cell aging and rejuvenation. Cell Stem Cell. 2012;10(5):520–30.
Florian MC, Nattamai KJ, Dörr K, Marka G, Überle B, Vas V, Eckl C, Andrä I, Schiemann M, Oostendorp RA. A canonical to non-canonical Wnt signalling switch in haematopoietic stem-cell ageing. Nature. 2013;503(7476):392–6.
Dong S, Wang Q, Kao Y-R, Diaz A, Tasset I, Kaushik S, Thiruthuvanathan V, Zintiridou A, Nieves E, Dzieciatkowska M. Chaperone-mediated autophagy sustains haematopoietic stem-cell function. Nature. 2021;591(7848):117–23.
Mohrin M, Shin J, Liu Y, Brown K, Luo H, Xi Y, Haynes CM, Chen D. A mitochondrial UPR-mediated metabolic checkpoint regulates hematopoietic stem cell aging. Science. 2015;347(6228):1374–7.
Brown K, Xie S, Qiu X, Mohrin M, Shin J, Liu Y, Zhang D, Scadden DT, Chen D. SIRT3 reverses aging-associated degeneration. Cell Rep. 2013;3(2):319–27.
Grigoryan A, Guidi N, Senger K, Liehr T, Soller K, Marka G, Vollmer A, Markaki Y, Leonhardt H, Buske C. LaminA/C regulates epigenetic and chromatin architecture changes upon aging of hematopoietic stem cells. Genome Biol. 2018;19(1):1–21.
Grigoryan A, Pospiech J, Krämer S, Lipka D, Liehr T, Geiger H, Kimura H, Mulaw MA, Florian MC. Attrition of x chromosome inactivation in aged hematopoietic stem cells. Stem Cell Rep. 2021;16(4):708–16.
Adelman ER, Huang H-T, Roisman A, Olsson A, Colaprico A, Qin T, Lindsley RC, Bejar R, Salomonis N, Grimes HL. Aging human hematopoietic stem cells manifest profound epigenetic reprogramming of enhancers that may predispose to leukemia. Cancer Discov. 2019;9(8):1080–101.
Mejia-Ramirez E, Florian MC. Understanding intrinsic hematopoietic stem cell aging. Haematologica. 2020;105(1):22.
Young K, Eudy E, Bell R, Loberg MA, Stearns T, Sharma D, Velten L, Haas S, Filippi M-D, Trowbridge JJ. Decline in IGF1 in the bone marrow microenvironment initiates hematopoietic stem cell aging. Cell Stem Cell. 2021;28(8):1473–82. e1477.
Cheng C-W, Adams GB, Perin L, Wei M, Zhou X, Lam BS, Da Sacco S, Mirisola M, Quinn DI, Dorff TB. Prolonged fasting reduces IGF-1/PKA to promote hematopoietic-stem-cell-based regeneration and reverse immunosuppression. Cell Stem Cell. 2014;14(6):810–23.
Matteini F, Mulaw MA, Florian MC. Aging of the hematopoietic stem cell niche: New tools to answer an old question. Front Immunol. 2021;12:738204.
Saçma M, Pospiech J, Bogeska R, de Back W, Mallm J-P, Sakk V, Soller K, Marka G, Vollmer A, Karns R. Haematopoietic stem cells in perisinusoidal niches are protected from ageing. Nat Cell Biol. 2019;21(11):1309–20.
Shen B, Tasdogan A, Ubellacker JM, Zhang J, Nosyreva ED, Du L, Murphy MM, Hu S, Yi Y, Kara N. A mechanosensitive peri-arteriolar niche for osteogenesis and lymphopoiesis. Nature. 2021;591(7850):438–44.
Schürch CM, Riether C, Ochsenbein AF. Cytotoxic CD8 + T cells stimulate hematopoietic progenitors by promoting cytokine release from bone marrow mesenchymal stromal cells. Cell Stem Cell. 2014;14(4):460–72.
Yamashita M, Passegué E. TNF-α coordinates hematopoietic stem cell survival and myeloid regeneration. Cell Stem Cell. 2019;25(3):357–72. e357.
Valletta S, Thomas A, Meng Y, Ren X, Drissen R, Sengül H, Di Genua C, Nerlov C. Micro-environmental sensing by bone marrow stroma identifies IL-6 and TGFβ1 as regulators of hematopoietic ageing. Nat Commun. 2020;11(1):4075.
Comazzetto S, Shen B, Morrison SJ. Niches that regulate stem cells and hematopoiesis in adult bone marrow. Dev Cell. 2021;56(13):1848–60.
Partridge L, Deelen J, Slagboom PE. Facing up to the global challenges of ageing. Nature. 2018;561(7721):45–56.
Geiger H, De Haan G, Florian MC. The ageing haematopoietic stem cell compartment. Nat Rev Immunol. 2013;13(5):376–89.
Rossi DJ, Jamieson CH, Weissman IL. Stems cells and the pathways to aging and cancer. Cell. 2008;132(4):681–96.
de Haan G, Lazare SS. Aging of hematopoietic stem cells. Blood J Am Soc Hematol. 2018;131(5):479–87.
Watt SM, Hua P, Roberts I. Increasing complexity of molecular landscapes in human hematopoietic stem and progenitor cells during development and aging. Int J Mol Sci. 2022;23(7):3675.
Su T-Y, Hauenstein J, Somuncular E, Dumral Ö, Leonard E, Gustafsson C, Tzortzis E, Forlani A, Johansson A-S, Qian H. Aging is associated with functional and molecular changes in distinct hematopoietic stem cell subsets. Nat Commun. 2024;15(1):7966.
Sun D, Luo M, Jeong M, Rodriguez B, Xia Z, Hannah R, Wang H, Le T, Faull KF, Chen R. Epigenomic profiling of young and aged HSCs reveals concerted changes during aging that reinforce self-renewal. Cell Stem Cell. 2014;14(5):673–88.
Kowalczyk MS, Tirosh I, Heckl D, Rao TN, Dixit A, Haas BJ, Schneider RK, Wagers AJ, Ebert BL, Regev A. Single-cell RNA-seq reveals changes in cell cycle and differentiation programs upon aging of hematopoietic stem cells. Genome Res. 2015;25(12):1860–72.
Itokawa N, Oshima M, Koide S, Takayama N, Kuribayashi W, Nakajima-Takagi Y, Aoyama K, Yamazaki S, Yamaguchi K, Furukawa Y. Epigenetic traits inscribed in chromatin accessibility in aged hematopoietic stem cells. Nat Commun. 2022;13(1):2691.
Somuncular E, Hauenstein J, Khalkar P, Johansson A-S, Dumral Ö, Frengen NS, Gustafsson C, Mocci G, Su T-Y, Brouwer H. CD49b identifies functionally and epigenetically distinct subsets of lineage-biased hematopoietic stem cells. Stem Cell Rep. 2022;17(7):1546–60.
Arai F, Stumpf PS, Ikushima YM, Hosokawa K, Roch A, Lutolf MP, Suda T, MacArthur BD. Machine learning of hematopoietic stem cell divisions from paired daughter cell expression profiles reveals effects of aging on self-renewal. Cell Syst. 2020;11(6):640–52. e645.
Bowie MB, Kent DG, Dykstra B, McKnight KD, McCaffrey L, Hoodless PA, Eaves CJ. Identification of a new intrinsically timed developmental checkpoint that reprograms key hematopoietic stem cell properties. Proceedings of the National Academy of Sciences 2007, 104(14):5878–5882.
Kovtonyuk LV, Fritsch K, Feng X, Manz MG, Takizawa H. Inflamm-aging of hematopoiesis, hematopoietic stem cells, and the bone marrow microenvironment. Front Immunol. 2016;7:502.
Müller-Sieburg CE, Cho RH, Thoman M, Adkins B, Sieburg HB. Deterministic regulation of hematopoietic stem cell self-renewal and differentiation. Blood J Am Soc Hematol. 2002;100(4):1302–9.
Haas S, Trumpp A, Milsom MD. Causes and consequences of hematopoietic stem cell heterogeneity. Cell Stem Cell. 2018;22(5):627–38.
Kim KM, Mura-Meszaros A, Tollot M, Krishnan MS, Gründl M, Neubert L, Groth M, Rodriguez-Fraticelli A, Svendsen AF, Campaner S. Taz protects hematopoietic stem cells from an aging-dependent decrease in PU. 1 activity. Nat Commun. 2022;13(1):5187.
Lui JC, Chen W, Barnes KM, Baron J. Changes in gene expression associated with aging commonly originate during juvenile growth. Mech Ageing Dev. 2010;131(10):641–9.
Nerlov C, Graf T. PU. 1 induces myeloid lineage commitment in multipotent hematopoietic progenitors. Genes Dev. 1998;12(15):2403–12.
Dakic A, Metcalf D, Di Rago L, Mifsud S, Wu L, Nutt SL. PU. 1 regulates the commitment of adult hematopoietic progenitors and restricts granulopoiesis. J Exp Med. 2005;201(9):1487–502.
Metcalf D, Dakic A, Mifsud S, Di Rago L, Wu L, Nutt S. Inactivation of PU. 1 in adult mice leads to the development of myeloid leukemia. Proceedings of the National Academy of Sciences 2006, 103(5):1486–1491.
Voncken JW, van Schaick H, Kaartinen V, Deemer K, Coates T, Landing B, Pattengale P, Dorseuil O, Bokoch GM, Groffen J. Increased neutrophil respiratory burst in bcr-null mutants. Cell. 1995;80(5):719–28.
Khoury H, Barbara M, Iscove N, Minden M. Differential expression of the BCR gene in sequential stages of murine hematopoietic hierarchy. Leukemia. 2011;25(4):711–3.
Greuber EK, Smith-Pearson P, Wang J, Pendergast AM. Role of ABL family kinases in cancer: from leukaemia to solid tumours. Nat Rev Cancer. 2013;13(8):559–71.
Cimmino L, Dawlaty MM, Ndiaye-Lobry D, Yap YS, Bakogianni S, Yu Y, Bhattacharyya S, Shaknovich R, Geng H, Lobry C. TET1 is a tumor suppressor of hematopoietic malignancy. Nat Immunol. 2015;16(6):653–62.
Joshi K, Zhang L, Breslin SJP, Kini AR, Zhang J. Role of TET dioxygenases in the regulation of both normal and pathological hematopoiesis. J Experimental Clin Cancer Res. 2022;41(1):294.
Blaney Davidson E, Scharstuhl A, Vitters E, Van Der Kraan P, Van Den Berg W. Reduced transforming growth factor-beta signaling in cartilage of old mice: role in impaired repair capacity. Arthritis Res therapy. 2005;7:1–10.
Loffredo FS, Steinhauser ML, Jay SM, Gannon J, Pancoast JR, Yalamanchi P, Sinha M, Dall’Osso C, Khong D, Shadrach JL. Growth differentiation factor 11 is a circulating factor that reverses age-related cardiac hypertrophy. Cell. 2013;153(4):828–39.
Yu Q, Stamenkovic I. Cell surface-localized matrix metalloproteinase-9 proteolytically activates TGF-β and promotes tumor invasion and angiogenesis. Genes Dev. 2000;14(2):163–76.
Imamura T, Takase M, Nishihara A, Oeda E, Hanai J-i, Kawabata M, Miyazono K. Smad6 inhibits signalling by the TGF-β superfamily. Nature. 1997;389(6651):622–6.
Narla A, Ebert BL. Ribosomopathies: human disorders of ribosome dysfunction. Blood J Am Soc Hematol. 2010;115(16):3196–205.
Curran SP, Ruvkun G. Lifespan regulation by evolutionarily conserved genes essential for viability. PLoS Genet. 2007;3(4):e56.
Grewal SS. Insulin/TOR signaling in growth and homeostasis: a view from the fly world. Int J Biochem Cell Biol. 2009;41(5):1006–10.
Hamilton B, Dong Y, Shindo M, Liu W, Odell I, Ruvkun G, Lee SS. A systematic RNAi screen for longevity genes in C. elegans. Genes Dev. 2005;19(13):1544–55.
Pan KZ, Palter JE, Rogers AN, Olsen A, Chen D, Lithgow GJ, Kapahi P. Inhibition of mRNA translation extends lifespan in Caenorhabditis elegans. Aging Cell. 2007;6(1):111–9.
Greer EL, Maures TJ, Hauswirth AG, Green EM, Leeman DS, Maro GS, Han S, Banko MR, Gozani O, Brunet A. Members of the H3K4 trimethylation complex regulate lifespan in a germline-dependent manner in C. elegans. Nature. 2010;466(7304):383–7.
Greer EL, Maures TJ, Ucar D, Hauswirth AG, Mancini E, Lim JP, Benayoun BA, Shi Y, Brunet A. Transgenerational epigenetic inheritance of longevity in Caenorhabditis elegans. Nature. 2011;479(7373):365–71.
Liu L, Cheung TH, Charville GW, Hurgo BMC, Leavitt T, Shih J, Brunet A, Rando TA. Chromatin modifications as determinants of muscle stem cell quiescence and chronological aging. Cell Rep. 2013;4(1):189–204.
Jeong M, Sun D, Luo M, Huang Y, Challen GA, Rodriguez B, Zhang X, Chavez L, Wang H, Hannah R. Large conserved domains of low DNA methylation maintained by Dnmt3a. Nat Genet. 2014;46(1):17–23.
Challen GA, Sun D, Jeong M, Luo M, Jelinek J, Berg JS, Bock C, Vasanthakumar A, Gu H, Xi Y. Dnmt3a is essential for hematopoietic stem cell differentiation. Nat Genet. 2012;44(1):23–31.
Ley TJ, Ding L, Walter MJ, McLellan MD, Lamprecht T, Larson DE, Kandoth C, Payton JE, Baty J, Welch J. DNMT3A mutations in acute myeloid leukemia. N Engl J Med. 2010;363(25):2424–33.
Yan X-J, Xu J, Gu Z-H, Pan C-M, Lu G, Shen Y, Shi J-Y, Zhu Y-M, Tang L, Zhang X-W. Exome sequencing identifies somatic mutations of DNA methyltransferase gene DNMT3A in acute monocytic leukemia. Nat Genet. 2011;43(4):309–15.
Grover A, Sanjuan-Pla A, Thongjuea S, Carrelha J, Giustacchini A, Gambardella A, Macaulay I, Mancini E, Luis TC, Mead A. Single-cell RNA sequencing reveals molecular and functional platelet bias of aged haematopoietic stem cells. Nat Commun. 2016;7(1):11075.
Martinez-Jimenez CP, Eling N, Chen H-C, Vallejos CA, Kolodziejczyk AA, Connor F, Stojic L, Rayner TF, Stubbington MJ, Teichmann SA. Aging increases cell-to-cell transcriptional variability upon immune stimulation. Science. 2017;355(6332):1433–6.
Mann M, Mehta A, de Boer C, Kowalczyk M, Lee K, Haldeman P, Rogel N, Knecht A, Farouq D, Regev A. Heterogeneous responses of hematopoietic stem cells to inflammatory stimuli are altered with age. Cell Rep. 2018;25:2992–3005. e5. In.
Ainciburu M, Ezponda T, Berastegui N, Alfonso-Pierola A, Vilas-Zornoza A, San Martin-Uriz P, Alignani D, Lamo-Espinosa J, San-Julian M, Jiménez-Solas T. Uncovering perturbations in human hematopoiesis associated with healthy aging and myeloid malignancies at single-cell resolution. Elife. 2023;12:e79363.
Steensma DP, Bejar R, Jaiswal S, Lindsley RC, Sekeres MA, Hasserjian RP, Ebert BL. Clonal hematopoiesis of indeterminate potential and its distinction from myelodysplastic syndromes. Blood J Am Soc Hematol. 2015;126(1):9–16.
Zink F, Stacey SN, Norddahl GL, Frigge ML, Magnusson OT, Jonsdottir I, Thorgeirsson TE, Sigurdsson A, Gudjonsson SA, Gudmundsson J. Clonal hematopoiesis, with and without candidate driver mutations, is common in the elderly. Blood J Am Soc Hematol. 2017;130(6):742–52.
Xie M, Lu C, Wang J, McLellan MD, Johnson KJ, Wendl MC, McMichael JF, Schmidt HK, Yellapantula V, Miller CA. Age-related mutations associated with clonal hematopoietic expansion and malignancies. Nat Med. 2014;20(12):1472–8.
Mayle A, Yang L, Rodriguez B, Zhou T, Chang E, Curry CV, Challen GA, Li W, Wheeler D, Rebel VI. Dnmt3a loss predisposes murine hematopoietic stem cells to malignant transformation. Blood. J Am Soc Hematol. 2015;125(4):629–38.
Busque L, Mio R, Mattioli J, Brais E, Blais N, Lalonde Y, Maragh M, Gilliland DG. Nonrandom X-inactivation patterns in normal females: lyonization ratios vary with age. 1996.
Champion KM, Gilbert JG, Asimakopoulos FA, Hinshelwood S, Green AR. Clonal haemopoiesis in normal elderly women: implications for the myeloproliferative disorders and myelodysplastic syndromes. Br J Haematol. 1997;97(4):920–6.
van den Akker EB, Pitts SJ, Deelen J, Moed MH, Potluri S, van Rooij J, Suchiman HED, Lakenberg N, De Dijcker WJ, Uitterlinden AG. Uncompromised 10-year survival of oldest old carrying somatic mutations in DNMT3A and TET2. Blood. J Am Soc Hematol. 2016;127(11):1512–5.
Hoehn RS, Jernigan PL, Chang AL, Edwards MJ, Pritts TA. Molecular mechanisms of erythrocyte aging. Biol Chem. 2015;396(6–7):621–31.
Fuster JJ, MacLauchlan S, Zuriaga MA, Polackal MN, Ostriker AC, Chakraborty R, Wu C-L, Sano S, Muralidharan S, Rius C. Clonal hematopoiesis associated with TET2 deficiency accelerates atherosclerosis development in mice. Science. 2017;355(6327):842–7.
Jaiswal S, Natarajan P, Silver AJ, Gibson CJ, Bick AG, Shvartz E, McConkey M, Gupta N, Gabriel S, Ardissino D. Clonal hematopoiesis and risk of atherosclerotic cardiovascular disease. N Engl J Med. 2017;377(2):111–21.
Rosendaal M, Adam J. Haemopoiesis by clonal succession? Blood Cells. 1984;10(2–3):473–85.
Roeder I, Kamminga LM, Braesel K, Dontje B, de Haan G, Loeffler M. Competitive clonal hematopoiesis in mouse chimeras explained by a stochastic model of stem cell organization. Blood. 2005;105(2):609–16.
Lu R, Neff NF, Quake SR, Weissman IL. Tracking single hematopoietic stem cells in vivo using high-throughput sequencing in conjunction with viral genetic barcoding. Nat Biotechnol. 2011;29(10):928–33.
Gerrits A, Dykstra B, Kalmykowa OJ, Klauke K, Verovskaya E, Broekhuis MJ, de Haan G, Bystrykh LV. Cellular barcoding tool for clonal analysis in the hematopoietic system. Blood J Am Soc Hematol. 2010;115(13):2610–8.
Sun J, Ramos A, Chapman B, Johnnidis JB, Le L, Ho Y-J, Klein A, Hofmann O, Camargo FD. Clonal dynamics of native haematopoiesis. Nature. 2014;514(7522):322–7.
Vionnie W, Yusuf RZ, Oki T, Wu J, Saez B, Wang X, Cook C, Baryawno N, Ziller MJ, Lee E. Epigenetic memory underlies cell-autonomous heterogeneous behavior of hematopoietic stem cells. Cell. 2016;167(5):1310–22. e1317.
Henninger J, Santoso B, Hans S, Durand E, Moore J, Mosimann C, Brand M, Traver D, Zon L. Clonal fate mapping quantifies the number of haematopoietic stem cells that arise during development. Nat Cell Biol. 2017;19(1):17–27.
Asahara T, Murohara T, Sullivan A, Silver M, van der Zee R, Li T, Witzenbichler B, Schatteman G, Isner JM. Isolation of putative progenitor endothelial cells for angiogenesis. Science. 1997;275(5302):964–6.
Fadini GP, Avogaro A. It is all in the blood: the multifaceted contribution of circulating progenitor cells in diabetic complications. Experimental Diabetes Research 2012, 2012.
Hazra S, Jarajapu Y, Stepps V, Caballero S, Thinschmidt J, Sautina L, Bengtsson N, Licalzi S, Dominguez J, Kern T. Long-term type 1 diabetes influences haematopoietic stem cells by reducing vascular repair potential and increasing inflammatory monocyte generation in a murine model. Diabetologia. 2013;56:644–53.
Orlandi A, Chavakis E, Seeger F, Tjwa M, Zeiher AM, Dimmeler S. Long-term diabetes impairs repopulation of hematopoietic progenitor cells and dysregulates the cytokine expression in the bone marrow microenvironment in mice. Basic Res Cardiol. 2010;105:703–12.
Busik JV, Tikhonenko M, Bhatwadekar A, Opreanu M, Yakubova N, Caballero S, Player D, Nakagawa T, Afzal A, Kielczewski J. Diabetic retinopathy is associated with bone marrow neuropathy and a depressed peripheral clock. J Exp Med. 2009;206(13):2897–906.
Ferraro F, Lymperi S, Méndez-Ferrer S, Saez B, Spencer JA, Yeap BY, Masselli E, Graiani G, Prezioso L, Rizzini EL. Diabetes impairs hematopoietic stem cell mobilization by altering niche function. Sci Transl Med. 2011;3(104):ra104101–104101.
Albiero M, Poncina N, Tjwa M, Ciciliot S, Menegazzo L, Ceolotto G, Vigili de Kreutzenberg S, Moura R, Giorgio M, Pelicci P. Diabetes causes bone marrow autonomic neuropathy and impairs stem cell mobilization via dysregulated p66Shc and Sirt1. Diabetes. 2014;63(4):1353–65.
Jha AK, Huang SC-C, Sergushichev A, Lampropoulou V, Ivanova Y, Loginicheva E, Chmielewski K, Stewart KM, Ashall J, Everts B. Network integration of parallel metabolic and transcriptional data reveals metabolic modules that regulate macrophage polarization. Immunity. 2015;42(3):419–30.
Lampropoulou V, Sergushichev A, Bambouskova M, Nair S, Vincent EE, Loginicheva E, Cervantes-Barragan L, Ma X, Huang SC-C, Griss T. Itaconate links inhibition of succinate dehydrogenase with macrophage metabolic remodeling and regulation of inflammation. Cell Metabol. 2016;24(1):158–66.
Chen L-L, Morcelle C, Cheng Z-L, Chen X, Xu Y, Gao Y, Song J, Li Z, Smith MD, Shi M. Itaconate inhibits TET DNA dioxygenases to dampen inflammatory responses. Nat Cell Biol. 2022;24(3):353–63.
Michelucci A, Cordes T, Ghelfi J, Pailot A, Reiling N, Goldmann O, Binz T, Wegner A, Tallam A, Rausell A. Immune-responsive gene 1 protein links metabolism to immunity by catalyzing itaconic acid production. Proceedings of the National Academy of Sciences 2013, 110(19):7820–7825.
Ghattas A, Griffiths HR, Devitt A, Lip GY, Shantsila E. Monocytes in coronary artery disease and atherosclerosis: where are we now? J Am Coll Cardiol. 2013;62(17):1541–51.
van der Vorst EP, Weber C. Novel features of monocytes and macrophages in cardiovascular biology and disease. Arteriosclerosis, thrombosis, and vascular biology 2019, 39(2):e30–7.
Robbins CS, Chudnovskiy A, Rauch PJ, Figueiredo J-L, Iwamoto Y, Gorbatov R, Etzrodt M, Weber GF, Ueno T, van Rooijen N. Extramedullary hematopoiesis generates Ly-6Chigh monocytes that infiltrate atherosclerotic lesions. Circulation. 2012;125(2):364–74.
Silvestre-Roig C, Braster Q, Ortega-Gomez A, Soehnlein O. Neutrophils as regulators of cardiovascular inflammation. Nat Reviews Cardiol. 2020;17(6):327–40.
Hilgendorf I, Gerhardt LM, Tan TC, Winter C, Holderried TA, Chousterman BG, Iwamoto Y, Liao R, Zirlik A, Scherer-Crosbie M. Ly-6Chigh monocytes depend on Nr4a1 to balance both inflammatory and reparative phases in the infarcted myocardium. Circul Res. 2014;114(10):1611–22.
Rasheed A. Niche Regulation of Hematopoiesis: The Environment Is Micro, but the Influence Is Large. Arteriosclerosis, thrombosis, and vascular biology 2022, 42(6):691–699.
Campaner S, Doni M, Verrecchia A, Fagà G, Bianchi L, Amati B. Myc, Cdk2 and cellular senescence: Old players, new game. Cell Cycle. 2010;9(18):3679–85.
Nayak G, Odaka Y, Prasad V, Solano AF, Yeo E-J, Vemaraju S, Molkentin JD, Trumpp A, Williams B, Rao S. Developmental vascular regression is regulated by a Wnt/β-catenin, MYC and CDKN1A pathway that controls cell proliferation and cell death. Development. 2018;145(12):dev154898.
Tikhonova AN, Dolgalev I, Hu H, Sivaraj KK, Hoxha E, Cuesta-Domínguez Á, Pinho S, Akhmetzyanova I, Gao J, Witkowski M. The bone marrow microenvironment at single-cell resolution. Nature. 2019;569(7755):222–8.
Jaiswal S, Fontanillas P, Flannick J, Manning A, Grauman PV, Mar BG, Lindsley RC, Mermel CH, Burtt N, Chavez A. Age-related clonal hematopoiesis associated with adverse outcomes. N Engl J Med. 2014;371(26):2488–98.
Dorsheimer L, Assmus B, Rasper T, Ortmann CA, Ecke A, Abou-El-Ardat K, Schmid T, Brüne B, Wagner S, Serve H. Association of mutations contributing to clonal hematopoiesis with prognosis in chronic ischemic heart failure. JAMA Cardiol. 2019;4(1):25–33.
Svensson EC, Madar A, Campbell CD, He Y, Sultan M, Healey ML, D’Aco K, Fernandez A, Wache-Mainier C, Ridker PM. TET2-driven clonal hematopoiesis predicts enhanced response to canakinumab in the CANTOS trial: an exploratory analysis. Circulation. 2018;138(Suppl1):A15111–15111.
Hoffmann J, Luxán G, Abplanalp WT, Glaser S-F, Rasper T, Fischer A, Muhly-Reinholz M, Potente M, Assmus B, John D. Post-myocardial infarction heart failure dysregulates the bone vascular niche. Nat Commun. 2021;12(1):3964.
Caiado F, Pietras EM, Manz MG. Inflammation as a regulator of hematopoietic stem cell function in disease, aging, and clonal selection. J Exp Med. 2021;218(7):e20201541.
Bick AG, Weinstock JS, Nandakumar SK, Fulco CP, Bao EL, Zekavat SM, Szeto MD, Liao X, Leventhal MJ, Nasser J. Inherited causes of clonal haematopoiesis in 97,691 whole genomes. Nature. 2020;586(7831):763–8.
Cook EK, Izukawa T, Young S, Rosen G, Jamali M, Zhang L, Johnson D, Bain E, Hilland J, Ferrone CK. Comorbid and inflammatory characteristics of genetic subtypes of clonal hematopoiesis. Blood Adv. 2019;3(16):2482–6.
Zhou L, McMahon C, Bhagat T, Alencar C, Yu Y, Fazzari M, Sohal D, Heuck C, Gundabolu K, Ng C. Reduced SMAD7 leads to overactivation of TGF-β signaling in MDS that can be reversed by a specific inhibitor of TGF-β receptor I kinase. Cancer Res. 2011;71(3):955–63.
Fenaux P, Kiladjian JJ, Platzbecker U. Luspatercept for the treatment of anemia in myelodysplastic syndromes and primary myelofibrosis. Blood. J Am Soc Hematol. 2019;133(8):790–4.
Arranz L, Sánchez-Aguilera A, Martín-Pérez D, Isern J, Langa X, Tzankov A, Lundberg P, Muntión S, Tzeng Y-S, Lai D-M. Neuropathy of haematopoietic stem cell niche is essential for myeloproliferative neoplasms. Nature. 2014;512(7512):78–81.
Kovtun I, von Bonin M, Ibneeva L, Frimmel J, Middeke JM, Kunadt D, Heberling L, Wobus M, Bornhäuser M, Grinenko T. Profound sympathetic neuropathy in the bone marrow of patients with acute myeloid leukemia. Leukemia. 2024;38(2):393–7.
Mistry JJ, Young KA, Trowbridge JJ. Bone Marrow Stromal Cell Senescence Induced By Dnmt3a-Mutant Hematopoietic Stem and Progenitor Cells Accelerates Clonal Hematopoiesis and Progression to Leukemia. Blood. 2022;140(Supplement 1):1265–1265.
Nguyen YT, Fujisawa M, Nguyen TB, Suehara Y, Sakamoto T, Matsuoka R, Abe Y, Fukumoto K, Hattori K, Noguchi M. Tet2 deficiency in immune cells exacerbates tumor progression by increasing angiogenesis in a lung cancer model. Cancer Sci. 2021;112(12):4931–43.
Abplanalp WT, Cremer S, John D, Hoffmann J, Schuhmacher B, Merten M, Rieger MA, Vasa-Nicotera M, Zeiher AM, Dimmeler S. Clonal hematopoiesis–driver DNMT3A mutations alter immune cells in heart failure. Circul Res. 2021;128(2):216–28.
Zioni N, Bercovich AA, Chapal-Ilani N, Bacharach T, Rappoport N, Solomon A, Avraham R, Kopitman E, Porat Z, Sacma M. Inflammatory signals from fatty bone marrow support DNMT3A driven clonal hematopoiesis. Nat Commun. 2023;14(1):2070.
Abegunde SO, Buckstein R, Wells RA, Rauh MJ. An inflammatory environment containing TNFα favors Tet2-mutant clonal hematopoiesis. Exp Hematol. 2018;59:60–5.
Liao M, Chen R, Yang Y, He H, Xu L, Jiang Y, Guo Z, He W, Jiang H, Wang J. Aging-elevated inflammation promotes DNMT3A R878H-driven clonal hematopoiesis. Acta Pharm Sinica B. 2022;12(2):678–91.
Boy M, Bisio V, Zhao L-P, Guidez F, Schell B, Lereclus E, Henry G, Villemonteix J, Rodrigues-Lima F, Gagne K. Myelodysplastic Syndrome associated TET2 mutations affect NK cell function and genome methylation. Nat Commun. 2023;14(1):588.
Winter S, Götze KS, Hecker JS, Metzeler KH, Guezguez B, Woods K, Medyouf H, Schäffer A, Schmitz M, Wehner R. Clonal hematopoiesis and its impact on the aging osteo-hematopoietic niche. Leukemia. 2024;38(5):936–46.
Download references
Acknowledgements
Our sincere thanks to department staff of Human Anatomy and Histology, Sechenov University, Moscow, Russia.
This study was supported by Herman Wells Pediatric Research Center, Indianapolis, USA.
Author information
Authors and affiliations.
Department of Oncology, I.M. Sechenov First Moscow State Medical University of the Ministry of Health of the Russian Federation (Sechenov University), 8/2 Trubetskaya Str, Moscow, 119991, Russia
Yang Xinyi & Reshetov Igor Vladimirovich
Department of Radiation Oncology, The First Affiliated Hospital of Zhengzhou University, No. 1, Jianshe East Road, Zhengzhou, 450000, China
Ruitai Fan & Junqi Liu
Department of Human Anatomy and Histology, I.M. Sechenov First Moscow State Medical University of the Ministry of Health of the Russian Federation (Sechenov University), 8/2 Trubetskaya Str, Moscow, 119991, Russia
Narasimha M. Beeraka & Vladimir N. Nikolenko
Raghavendra Institute of Pharmaceutical Education and Research (RIPER), Anantapuramu, Chiyyedu, Andhra Pradesh, 515721, India
Narasimha M. Beeraka & Padmanabha Reddy Y
Herman B. Wells Center for Pediatric Research, Department of Pediatrics, Indiana University School of Medicine, 1044 W. Walnut Street, R4-168, Indianapolis, IN, 46202, USA
Narasimha M. Beeraka & Dinisha Kamble
Department of Computer Science, St Philomena’s College (Autonomous), Bangalore - Mysore Rd, Bannimantap, Mysuru, Karnataka, 570015, India
Allaka Naga Lakshmi
Laboratory of Chemical Biology, Department of Studies in Organic Chemistry, University of Mysore, Mysore, Karnataka, 570006, India
Basappa Basappa
Department of Chemistry, Faculty of science, Dr B R Ambedkar Open University, Wanaparthy, Telangana, 509103, India
Allaka Satyavathi
Department of Studies in Molecular Biology, Faculty of Science and Technology, University of Mysore, Mysore, Karnataka, 570006, India
Narasimha M. Beeraka
You can also search for this author in PubMed Google Scholar
Contributions
Yang Xinyi (YX), Reshetov Igor Vladimirovich (RIV), Narasimha M Beeraka (NMB), Allaka Satyavathi (AS), Dinisha Kamble (DK), Vladimir N Nikolenko (VNN), Allaka Naga Lakshmi (ANL), Basappa Basappa (BB), Padmanabha Reddy Y (PRY), Ruitai Fan (RF), Junqi Liu (JL) designed the concept, and YX (Primary author) NMB (Main corresponding author) and RF wrote the manuscript; NMB, YX proofread, edited, and analyzed the content of the article. All authors reviewed the manuscript and approved it before submission.
Corresponding authors
Correspondence to Narasimha M. Beeraka or Ruitai Fan .
Ethics declarations
Ethics approval, consent for publication, conflict of interest.
The authors declare no conflict of interest.
Additional information
Publisher’s note.
Springer Nature remains neutral with regard to jurisdictional claims in published maps and institutional affiliations.
Rights and permissions
Open Access This article is licensed under a Creative Commons Attribution-NonCommercial-NoDerivatives 4.0 International License, which permits any non-commercial use, sharing, distribution and reproduction in any medium or format, as long as you give appropriate credit to the original author(s) and the source, provide a link to the Creative Commons licence, and indicate if you modified the licensed material. You do not have permission under this licence to share adapted material derived from this article or parts of it. The images or other third party material in this article are included in the article’s Creative Commons licence, unless indicated otherwise in a credit line to the material. If material is not included in the article’s Creative Commons licence and your intended use is not permitted by statutory regulation or exceeds the permitted use, you will need to obtain permission directly from the copyright holder. To view a copy of this licence, visit http://creativecommons.org/licenses/by-nc-nd/4.0/ .
Reprints and permissions
About this article
Cite this article.
Xinyi, Y., Vladimirovich, R.I., Beeraka, N.M. et al. Emerging insights into epigenetics and hematopoietic stem cell trafficking in age-related hematological malignancies. Stem Cell Res Ther 15 , 401 (2024). https://doi.org/10.1186/s13287-024-04008-4
Download citation
Received : 31 July 2024
Accepted : 22 October 2024
Published : 06 November 2024
DOI : https://doi.org/10.1186/s13287-024-04008-4
Share this article
Anyone you share the following link with will be able to read this content:
Sorry, a shareable link is not currently available for this article.
Provided by the Springer Nature SharedIt content-sharing initiative
- Hematological malignancies
- Epigenetics
- Clonal hematopoiesis
- Signaling pathways
Stem Cell Research & Therapy
ISSN: 1757-6512
- Submission enquiries: Access here and click Contact Us
- General enquiries: [email protected]

DNA RNA and Cells
GIOSTAR Announces FDA Clearance of the IND for Starting PHASE-2 Clinical Trial for Developing Specific Stem Cell Therapy for Type II Diabetes.
Published on: Wednesday, 13 November 2024 05:23 PM
SAN DIEGO, CA, USA I November 13, 2024 I Global Institute of Stem Cell Therapy and Research, Inc. known as GIOSTAR , a San Diego, California based Global organization at the forefront of stem cell research over two decades, developing a novel cellular therapy pipeline to improve the standard of care for treating Type II diabetes patients, today announced that the United States Food and Drug Administration (FDA) has cleared its investigational new drug (IND) application to start a Phase–2 clinical trial for DT2-SCT. The Company’s novel approach using autologous mesenchymal stem cells to alleviate the disease-caused damage in diabetics offers a new hope to address the sufferings in diabetes patients without much side-effects.
Diabetes is not just a disease but a global health crisis. It is estimated to affect more than one billion people worldwide. The financial burden on the global healthcare system to treat diabetes is expected to reach more than one trillion dollars annually. GIOSTAR CEO, President, and Cofounder Mr. Deven Patel stated, “Upon a successful completion of the clinical trials, GIOSTAR intends to make this treatment affordable to masses and poised to capture significant global market share due to GIOSTAR’s existing global infrastructure of hospitals and research centers.”
According to the Chairman and Cofounder of GIOSTAR, Dr. Anand Srivastava , “DT2-SCT is a cellular therapy for Type II diabetics which uses autologous stem cells, isolated from the visceral tissues of the recipients, developed to target systemic ill-effects caused by diabetes-induced pathology in patients. We are pleased to reach this milestone following extensive research and development.”
GIOSTAR expects to complete the Phase-2 clinical trial using the DT2-SCT in Type II diabetics within 12 to 18 months. GIOSTAR anticipates enrolling participants for the study at few sites across the United States.
“The diabetes is now considered as a lifestyle disease. In many cases, even lifestyle changes are not enough to eliminate the risk of developing diabetes due to certain genetic risk factors,” stated Patel . “Our innovative and noninvasive stem cell-based therapeutics may offer better treatment option for diabetic patients.”
About: ( GIOSTAR )
GIOSTAR is a global stem cell research organization committed to the discovery, development, and commercialization of stem cell based treatments to make a meaningful difference in the lives of people impacted by difficult-to-treat degenerative and other diseases. The company also aims to develop stem cell-based therapies for arthritis, long COVID complications , cancer vaccines and the fairly uncommon area of making red blood cells from stem cells. GIOSTAR red blood cell technology is ready to scale and preparing its IND for US FDA. The leadership of GIOSTAR aims to make these stem cell treatments available to the masses at affordable prices.
SOURCE: GIOSTAR
Custom Report
La Merie Publishing offers the service of preparing customized reports tailored to the needs and specifications of the customer. We conduct scientific & medical literature evaluations for clients of the biopharmaceutical industry within the scope of our expertise. Target or technology pipeline and corporate benchmark analysis and assessment reports can be prepared according to the specifications of the client from the pharmaceutical or biotechnology industry.
Featured Reports
La Merie Biologics
La Merie Publishing releases a weekly newsletter with a focus on biopharmaceutical research and development.
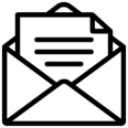
2023 Sales of Antibodies & Proteins
New product alert.
La Merie Publishing offers an e-mail notification service about the release of new products of La Merie Publishing. This New Product Release Alert also informs about the release of FREE reports produced by La Merie Publishing.
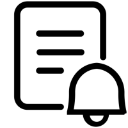
RElated News
- Personal Finance
- Today's Paper
- Partner Content
- Web Stories
- Entertainment
- Social Viral
Over 800 million adults have diabetes globally, many untreated: Study
In 2022, there were around 828 million people aged 18 years and older with type 1 and type 2 diabetes worldwide, the study found.
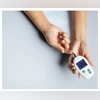)
Photo: Shutterstock.com
Listen to This Article
More from this section.
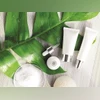)
Top 5 winter skincare routines for your dry skin to keep it healthy
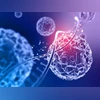)
ICMR seeks 'out of the box' ideas from scientists to solve health problems
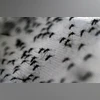)
Delhi reports 5-year high in chikungunya, malaria cases; dengue declines
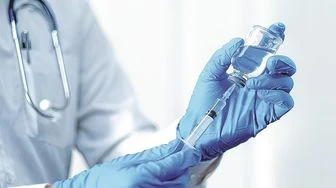)
India needs to expand local vaccine manufacturing, research: GTRI report
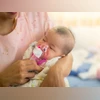)
World Pneumonia Day 2024: Know history, prevention, significance and theme
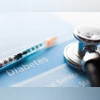)
World Diabetes Day 2024: Theme, history and best winter foods for patients
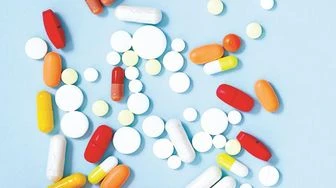)
Wockhardt gains 4% on filing for DCGI's approval for its insulin injection
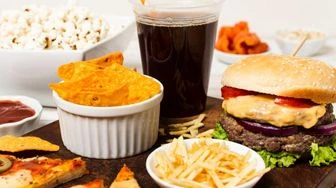)
How fried and processed foods are fuelling India's diabetes epidemic
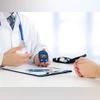)
Glucose Tolerance Test: Here's why and when your doctor recommends it
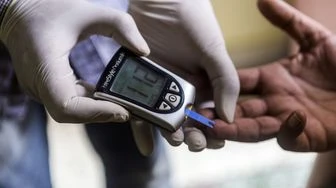)
Breakthrough stem-cell transplant in China offers hope for Type 1 diabetes
Don't miss the most important news and views of the day. Get them on our Telegram channel
First Published: Nov 14 2024 | 5:19 PM IST
Explore News
- Suzlon Energy Share Price Adani Enterprises Share Price Adani Power Share Price IRFC Share Price Tata Motors Share Price Tata Steel Share Price Yes Bank Share Price Infosys Share Price SBI Share Price Reliance shares
- Latest News Company News Market News India News Politics News Cricket News Personal Finance Technology News World News Industry News Education News Opinion Shows Economy News Lifestyle News Health News
- Today's Paper About Us T&C Privacy Policy Cookie Policy Disclaimer Investor Communication GST registration number List Compliance Contact Us Advertise with Us Sitemap Subscribe Careers BS Apps
- Maharashtra Elections 2024 Business Standard at 50 IPO News Business Standard BFSI Summit 2024 Jharkhand Elections Results 2024 US Election 2024


Nanotherapy offers new hope for the treatment of Type 1 diabetes
Individuals living with Type 1 diabetes must carefully follow prescribed insulin regimens every day, receiving injections of the hormone via syringe, insulin pump or some other device. And without viable long-term treatments, this course of treatment is a lifelong sentence.
Pancreatic islets control insulin production when blood sugar levels change, and in Type 1 diabetes, the body’s immune system attacks and destroys such insulin-producing cells. Islet transplantation has emerged over the past few decades as a potential cure for Type 1 diabetes. With healthy transplanted islets, Type 1 diabetes patients may no longer need insulin injections, but transplantation efforts have faced setbacks as the immune system continues to eventually reject new islets. Current immunosuppressive drugs offer inadequate protection for transplanted cells and tissues and are plagued by undesirable side effects.
Now a team of researchers at Northwestern University led by SQI members Evan Scott and Guillermo Ameer has discovered a technique to help make immunomodulation more effective. The method uses nanocarriers to re-engineer the commonly used immunosuppressant rapamycin. Using these rapamycin-loaded nanocarriers, the researchers generated a new form of immunosuppression capable of targeting specific cells related to the transplant without suppressing wider immune responses. The paper was published Jan. 17 in the journal Nature Nanotechnology .
Specifying the body’s attack
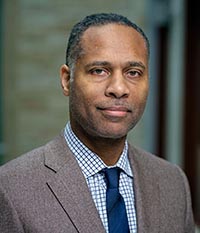
Ameer has been working on improving the outcomes of islet transplantation by providing islets with an engineered environment, using biomaterials to optimize their survival and function. However, problems associated with traditional systemic immunosuppression remain a barrier to the clinical management of patients and must also be addressed to truly have an impact on their care, said Ameer, the Daniel Hale Williams Professor of Biomedical Engineering at Northwestern’s McCormick School of Engineering and Professor of Surgery at Feinberg School of Medicine . Ameer also serves as the director of the Center for Advanced Regenerative Engineering (CARE) .
“This was an opportunity to partner with Evan Scott, a leader in immunoengineering, and engage in a convergence research collaboration that was well executed with tremendous attention to detail by Jacqueline Burke, a National Science Foundation Graduate Research Fellow,” Ameer said.
Rapamycin is well-studied and commonly used to suppress immune responses during other types of treatment and transplants, notable for its wide range of effects on many cell types throughout the body. Typically delivered orally, rapamycin’s dosage must be carefully monitored to prevent toxic effects. Yet, at lower doses it has poor effectiveness in cases such as islet transplantation.
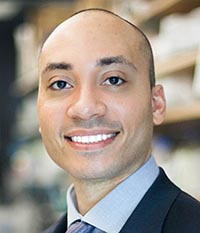
Scott, also a member of CARE, said he wanted to see how the drug could be enhanced by putting it in a nanoparticle and “controlling where it goes within the body.”
“To avoid the broad effects of rapamycin during treatment, the drug is typically given at low dosages and via specific routes of administration, mainly orally,” said Scott, the Kay Davis Professor and an associate professor of biomedical engineering at McCormick and microbiology-immunology at Feinberg. “But in the case of a transplant, you have to give enough rapamycin to systemically suppress T cells, which can have significant side effects like hair loss, mouth sores and an overall weakened immune system.”
Following a transplant, immune cells, called T cells, will reject newly introduced foreign cells and tissues. Immunosuppressants are used to inhibit this effect but can also impact the body’s ability to fight other infections by shutting down T cells across the body. But the team formulated the nanocarrier and drug mixture to have a more specific effect. Instead of directly modulating T cells — the most common therapeutic target of rapamycin — the nanoparticle would be designed to target and modify antigen presenting cells (APCs) that allow for more targeted, controlled immunosuppression.
Using nanoparticles also enabled the team to deliver rapamycin through a subcutaneous injection, which they discovered uses a different metabolic pathway to avoid extensive drug loss that occurs in the liver following oral administration. This route of administration requires significantly less rapamycin to be effective — about half the standard dose.
“We wondered, can rapamycin be re-engineered to avoid non-specific suppression of T cells and instead stimulate a tolerogenic pathway by delivering the drug to different types of immune cells?” Scott said. “By changing the cell types that are targeted, we actually changed the way that immunosuppression was achieved.”
A ‘pipe dream’ come true in diabetes research
The team tested the hypothesis on mice, introducing diabetes to the population before treating them with a combination of islet transplantation and rapamycin, delivered via the standard Rapamune® oral regimen and their nanocarrier formulation. Beginning the day before transplantation, mice were given injections of the altered drug and continued injections every three days for two weeks.
The team observed minimal side effects in the mice and found the diabetes was eradicated for the length of their 100-day trial; but the treatment should last the transplant’s lifespan. The team also demonstrated the population of mice treated with the nano-delivered drug had a “robust immune response” compared to mice given standard treatments of the drug.
The concept of enhancing and controlling side effects of drugs via nanodelivery is not a new one, Scott said. “But here we’re not enhancing an effect, we are changing it – by repurposing the biochemical pathway of a drug, in this case mTOR inhibition by rapamycin, we are generating a totally different cellular response.”
The team’s discovery could have far-reaching implications. “This approach can be applied to other transplanted tissues and organs, opening up new research areas and options for patients,” Ameer said. “We are now working on taking these very exciting results one step closer to clinical use.”
Jacqueline Burke, the first author on the study and a National Science Foundation Graduate Research Fellow and researcher working with Scott and Ameer at CARE, said she could hardly believe her readings when she saw the mice’s blood sugar plummet from highly diabetic levels to an even number. She kept double-checking to make sure it wasn’t a fluke, but saw the number sustained over the course of months.
Research hits close to home
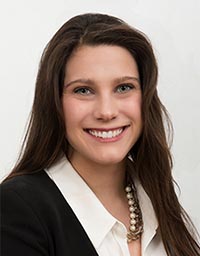
For Burke, a doctoral candidate studying biomedical engineering, the research hits closer to home. Burke is one such individual for whom daily shots are a well-known part of her life. She was diagnosed with Type 1 diabetes when she was nine, and for a long time knew she wanted to somehow contribute to the field.
“At my past program, I worked on wound healing for diabetic foot ulcers, which are a complication of Type 1 diabetes,” Burke said. “As someone who’s 26, I never really want to get there, so I felt like a better strategy would be to focus on how we can treat diabetes now in a more succinct way that mimics the natural occurrences of the pancreas in a non-diabetic person.”
The all-Northwestern research team has been working on experiments and publishing studies on islet transplantation for three years, and both Burke and Scott say the work they just published could have been broken into two or three papers. What they’ve published now, though, they consider a breakthrough and say it could have major implications on the future of diabetes research.
Scott has begun the process of patenting the method and collaborating with industrial partners to ultimately move it into the clinical trials stage. Commercializing his work would address the remaining issues that have arisen for new technologies like Vertex’s stem-cell derived pancreatic islets for diabetes treatment.
The paper, “Subcutaneous nanotherapy repurposes the immunosuppressive mechanism of rapamycin to enhance allogeneic islet graft viability,” was supported by the National Science Foundation (Award no. 1453576), the National Institute of Health Director’s New Innovator Award (NHLBI 1DP2HL132390-01), and the Center for Advanced Regenerative Engineering.
This article first appeared on Northwestern Now .

IMAGES
COMMENTS
Keywords: Type 2 diabetes mellitus (T2DM), type 1 diabetes mellitus (T1DM), stem cells, glycosylated hemoglobin (HbA1C) Introduction. Diabetes mellitus (DM) is a major health problem and the leading cause of death in the world; it is particularly responsible for 4 million deaths per year .
To accurately identify clinical trials of stem cell transplantation in DM patients, a search was performed using a combination of medical subject heading (MeSH) terms and text words: "diabetes mellitus, type 1" or "diabetes mellitus, type 2" and "stem cell transplantation" and "therapy" or "therapeutic use." Inclusion and Exclusion Criteria
According to a report from the International Diabetes Federation, there were 424.9 million diabetic patients aged 20-79 years worldwide in 2017, and the number is expected to reach 628.6 million by 2045 [].In addition, the number of children and adolescents (0-19 years old) with type 1 diabetes has reached 1.1 million [].Diabetes mellitus (DM), including type 1 DM (T1DM) and type 2 DM (T2DM ...
For Harvard Stem Cell Institute Co-Director and Xander University Professor Douglas Melton, whose lab pioneered the science behind the therapy, the trial marked the most recent turning point in a decades-long effort to understand and treat the disease. In a conversation with the Gazette, Melton discussed the science behind the advance, the ...
A 25-year-old woman with type 1 diabetes started producing her own insulin less than three months after receiving a transplant of reprogrammed stem cells 1. She is the first person with the ...
Diabetes mellitus (DM), a chronic metabolic disease, poses a significant global health challenge, with current treatments often fail to prevent the long-term disease complications. Mesenchymal stem/stromal cells (MSCs) are, adult progenitors, able to repair injured tissues, exhibiting regenerative effects and immunoregulatory and anti-inflammatory responses, so they have been emerged as a ...
This article reviews the progress and challenges of generating insulin-producing cells from human embryonic and pluripotent stem cells for type 1 diabetes. It highlights the key papers and discoveries that have advanced the field, as well as the remaining issues to be resolved before a clinical application.
SAN DIEGO--(BUSINESS WIRE)--Global Institute of Stem Cell Therapy and Research, Inc. known as GIOSTAR, a San Diego, California based Global organization at the forefront of stem cell research over two decades, developing a novel cellular therapy pipeline to improve the standard of care for treating Type II diabetes patients, today announced that the United States Food and Drug Administration ...
Lessons learned. Autologous bone marrow-derived mesenchymal stem cell administration exhibited a reduction in HbA1c and fasting blood glucose level in patients with a type 2 diabetes mellitus (T2DM) duration of <10 years and a body mass index of <23, and the effectiveness appeared to diminish after 6 months.
This systematic literature review aims to compare the efficacy and safety of traditional and stem cell (SC) therapies for type 1 (T1DM) and type 2 (T2DM) diabetes mellitus patients. Methods The PubMed, SciELO, BVS, and Medline databases were searched, and 38 original articles were selected, which included 647 control cases and 654 treatments ...
In general, DM can be mainly divided into type 1 diabetes mellitus (T1DM) and type 2 diabetes mellitus (T2DM). T1DM has been identified as an autoimmune disease in which T cells attack insulin-secreting β cells [7], whereas in T2DM, dysfunction of β cells has been regarded as integral to the pathogenesis of the disease. Available functional ...
Sun X, Hao H, Han Q, et al. Human umbilical cord-derived mesenchymal stem cells ameliorate insulin resistance by suppressing NLRP3 inflammasome-mediated inflammation in type 2 diabetes rats. Stem Cell Res Ther. 2017 Nov 2;8 (open in a new window) (1 (open in a new window)):241. doi: 10.1186/s13287-017-0668-1
This makes them the most commonly used adult stem cells and ideal candidates to treat diabetes. Objective: To assess the safety and efficacy of mesenchymal stem cells (MSCs) in treating Type 2 diabetes (T2D) in humans. Methods: Mesenchymal stem cell-based treatments were studied in 262 patients. A total of 6 out of 58 trials fit our inclusion ...
Restoration of insulin independence and normoglycemia has been the overarching goal in diabetes research and therapy. While whole-organ and islet transplantation have become gold-standard procedures in achieving glucose control in diabetic patients, the profound lack of suitable donor tissues severely hampers the broad application of these therapies.
3 Broad Center of Regeneration Medicine and Stem Cell Research, University of California, San Francisco, San Francisco, CA 94143, USA. ... Antithymocyte globulin therapy for patients with recent-onset type 1 diabetes: 2 year results of a randomised trial. Diabetologia. 2016; 59:1153-1161. Crossref.
Type 2 diabetes (T2D) typically starts with insulin resistance in peripheral tissues and proceeds with gradual loss of islet function due to the reduction in β-cell mass or dedifferentiation of ...
The first-in-human phase 1 clinical trial of autologous transplantation of chemically induced pluripotent stem-cell-derived islets for type 1 diabetes treatment was published on Sept 25, 2024. The patient, a 25-year-old woman, had sustained insulin independence starting 75 days post-transplantation and remained insulin-independent for the follow-up period of 1 year, with a decrease in HbA 1c ...
St. Petersburg, Fla. - September 12, 2023 - Scientists at Johns Hopkins All Children's Hospital, along with an international team of researchers, are shedding new light on the causes of Type 2 diabetes. The new research, published in the journal Nature Communications, offers a potential strategy for developing new therapies that could restore dysfunctional pancreatic beta-cells or ...
Abstract. Since the discovery of insulin a century ago, insulin injection has been a primary treatment for both type 1 (T1D) and type 2 diabetes (T2D). T2D is a complicated disea se that is triggered by the dysfunction of insulin-producing β cells and insulin resistance in peripheral tissues. Insulin injection partially compensates for the ...
In a new study, published in Cell, a 25-year-old woman with type 1 diabetes became the first person in the world to receive a stem cell transplant created from her own cells. Scientists hope that this approach could reduce or eliminate the need for immunosuppressants. Researchers at Peking University, Beijing, extracted cells from the ...
Abstract. Objective: To compile and analyze the published studies on cell therapy for type 2 diabetes mellitus (T2DM) to obtain a better insight into management of T2DM that involved stem cell therapy. Methods: We searched all published studies in Pubmed/Medline, and Cochrane library, using keywords: 'stem cell' AND 'therapy' AND ...
Background Type 1 diabetes, an autoimmune disorder leading to the destruction of pancreatic β-cells, requires lifelong insulin therapy. Islet transplantation offers a promising solution but faces challenges such as limited availability and the need for immunosuppression. Induced pluripotent stem cells (iPSCs) provide a potential alternative source of functional β-cells and have the ...
VX-264 is an investigational cell therapy in which allogeneic human stem cell-derived islets are encapsulated in a channel array device designed to shield the cells from the body's immune system. VX-264 is designed to be surgically implanted and is currently being evaluated for patients with T1D. About the VX-264 Phase 1/2 Clinical Trial
After this success, the method opened a new field in stem cell research with a generation of iPSC lines that can be customized and biocompatible with the patient. Recently, studies have focused on reducing carcinogenesis and improving the conduction system. ... In the case of type 1 diabetes, insulin-producing cells in the pancreas are ...
Islet cell transplantation has a quarter-century track record of success in patients with type 1 diabetes mellitus (T1DM) who have recurrent hypoglycaemia or glycaemic lability 1,2,3.Islets are ...
Background Hematopoiesis within the bone marrow (BM) is a complex and tightly regulated process predominantly influenced by immune factors. Aging, diabetes, and obesity are significant contributors to BM niche damage, which can alter hematopoiesis and lead to the development of clonal hematopoiesis of intermediate potential (CHIP). Genetic/epigenetic alterations during aging could influence BM ...
SAN DIEGO, CA, USA I November 13, 2024 I Global Institute of Stem Cell Therapy and Research, Inc. known as GIOSTAR, a San Diego, California based Global organization at the forefront of stem cell research over two decades, developing a novel cellular therapy pipeline to improve the standard of care for treating Type II diabetes patients, today announced that the United States Food and Drug ...
In 2022, there were around 828 million people aged 18 years and older with type 1 and type 2 diabetes worldwide, the study found. Among adults aged 30 years and older, 445 million, or 59% of them, were not receiving treatment, the authors said.
Pancreatic islets control insulin production when blood sugar levels change, and in Type 1 diabetes, the body's immune system attacks and destroys such insulin-producing cells. Islet transplantation has emerged over the past few decades as a potential cure for Type 1 diabetes.